8 NERVOUS TISSUE
DEVELOPMENT OF THE NERVOUS SYSTEM
The CNS develops from the primitive ectoderm (Figure 8-1 and Box 8-A). A simple epithelial disk—the neural plate—rapidly rolls into a hollow cylinder—the neural tube. This process is known as neurulation. During this process, a specialized portion of the neural plate—the neural crest—separates from both the neural tube and the overlying ectoderm. In later development, the neural crest forms the neurons of the peripheral ganglia and other components of the PNS. A defect in the closing of the neural tube causes different congenital malformations (see Box 8-B).
Box 8-A Three sources of the CNS
Box 8-B Neural tube defects
Some of these cells invade developing visceral organs and form the parasympathetic and enteric ganglia and the chromaffin cells of the adrenal medulla.
The early neural tube consists of a pseudostratified columnar epithelium formed by three zones (Figure 8-2): (1) the ventricular zone—the zone where progenitor cells give rise to most cells of the nervous tissue (except microglial cells); (2) the intermediate zone—where neurons migrate toward the cortical plate and where excess neurons are destroyed by apoptosis; and (3) the cortical plate—the future gray matter of the cerebral cortex.
Immature neurons leave the ventricular zone, migrate to the intermediate zone, lose their capacity to undergo cell division, and differentiate into functional neurons. The neuronal migration mechanism and the consequences of abnormal migration are highlighted in Box 8-C.
Box 8-C Neuronal migration
During this differentiation process, a selection process—similar to that in the thymus for T cells (see Chapter 10, Immune-Lymphatic System)—results in either neuronal heterogeneity or death. Neurons that become postmitotic in the intermediate zone reach the outer layers of the cortical plate and continue their differentiation.
Once the production of immature neurons is complete, the germinal or ventricular cells produce glioblasts, which differentiate into astrocytes, oligodendrocytes, and ependymoblasts. Ependymoblasts give rise to ependymal cells, lining the ventricular cavities of the CNS, and choroid epithelial cells, which are components of the choroid plexus.
CELL TYPES: NEURONS AND GLIA
Neuron
The functional unit of the nervous system is a highly specialized, excitable cell, the nerve cell or neuron. Neurons usually consist of three principal components (Figures 8-3 and 8-4): (1) soma or cell body, (2) dendrites, and (3) axon.
The dendrites are processes that arise as multiple treelike branches of the soma, forming a dendritic tree collectively. The entire surface of the dendritic branches is covered by small protrusions called dendritic spines. Dendritic spines establish numerous axonal synaptic connections, as we will see later (see Figure 8-7).
Types of neurons
Different types of neurons can be identified on the basis of the number and length of processes emerging from the soma (Figure 8-5):
According to the number of processes, neurons can be classified as:
Based on the length of the axon relative to the dendritic tree, multipolar neurons can be subclassified into (1) Golgi type I neurons, when the axon extends beyond the limits of the dendritic tree and (2) Golgi type II neurons, when an axon terminates in the immediate area of the cell body and does not extend beyond the limits of the dendritic tree. Small stellate cells of the cerebral cortex are Golgi type II cells.
Designation of groups of neurons and axons
Clusters of neurons arranged in a layer form a stratum or lamina (cerebral cortex). When neurons form longitudinal groups, these groups are designated columns.
Synaptic terminals and synapses
The synaptic terminal (Figure 8-6) is specialized for the transmission of a chemical message in response to an action potential. The synapse is the junction between the presynaptic terminal of an axon and a postsynaptic membrane receptor surface, generally a dendrite.
Presynaptic terminals contain a large number of membrane-bound vesicles (40 to 100 nm in diameter), the synaptic vesicles. Synaptic vesicles originate in the neuronal soma and are transported by molecular motor proteins along the axon (axonal transport) (Figure 8-7). Each vesicle contains a neurotransmitter. Presynaptic terminals contain mitochondria, components of the smooth endoplasmic reticulum, microtubules, and a few neurofilaments.
Synapses are classified by their location on the postsynaptic neuron (Figure 8-8) as follows:
Clinical significance: Axonal transport of rabies virus
The role of the axonal cytoskeleton and motor proteins (kinesin and cytoplasmic dynein; see Figure 8-7) was discussed in the Cytoskeleton section of Chapter 1, Epithelium. We emphasize once more the bidirectional transport of molecules along the axon: kinesin-mediated anterograde axonal transport of neurotransmitters—from the cell body toward the axon terminals, and the cytoplasmic dynein-mediated retrograde axonal transport of growth factors and recycling of axon terminal components—from the axon terminals to the cell body (see Box 8-D).
Box 8-D Neurotransmitters
Axonal transport is important in the pathogenesis of neurologic infectious diseases. For example, the rabies virus introduced by the bite of a rabid animal replicates in the muscle tissue from as little as 2 to 16 weeks or longer. After binding to the acetylcholine receptor, the viral particles are mobilized by retrograde axonal transport to the cell body of neurons supplying the affected muscle. The rabies virus continues to replicate within infected neurons and after the shedding of the virions by budding, they are internalized by the terminals of adjacent neurons. Further dissemination of the rabies virus occurs in the CNS. From the CNS, the rabies virus is transported by anterograde axonal transport by the peripheral nerves to the salivary glands. The virus enters the saliva to be transmitted by the bite. Painful spasm of the throat muscles on swallowing accounts for hydrophobia (aversion to swallowing water).
Astrocytes
Fibrous astrocytes are found predominantly in white matter and have long thin processes with few branches. Protoplasmic astrocytes reside predominantly in gray matter and have shorter processes with many short branches. Astrocytic processes end in expansions called end-feet (Figure 8-9).
One of the distinctive features of astrocytes is the presence of a large number of glial filaments (glial fibrillary acidic protein, a class of intermediate filament studied in Chapter 1, Epithelium). Glial fibrillary acidic protein is a valuable marker for the identification of astrocytes by immunohistochemistry. Nuclei of astrocytes are large, ovoid, and lightly stained.
Most brain capillaries and the inner surface of the pia mater are completely surrounded by astrocytic end-feet (see Figure 8-9) forming the glia limitans (also called the glial limiting membrane). The close association of astrocytes and brain capillaries suggests a role in the regulation of brain metabolism.
Astrocytes surround neurons and neuronal processes in areas devoid of myelin sheaths and form the structural matrix for the nervous system.
Oligodendrocytes and Schwann cells: Myelinization
Processes of oligodendrocytes envelop axons and form a sheathlike covering (Figure 8-10). The formation of this sheath is similar to that of Schwann cells in peripheral nerves.
Myelin sheaths extend from the initial segments of axons to their terminal branches. The segments of myelin formed by individual oligodendrocyte processes are internodes. The periodic gaps between the internodes are the nodes of Ranvier.
During the formation of the myelin sheath, a cytoplasmic process of the oligodendrocyte wraps around the axon and, after one full turn, the external surface of the glial membrane makes contact with itself, forming the inner mesaxon (Figure 8-11).
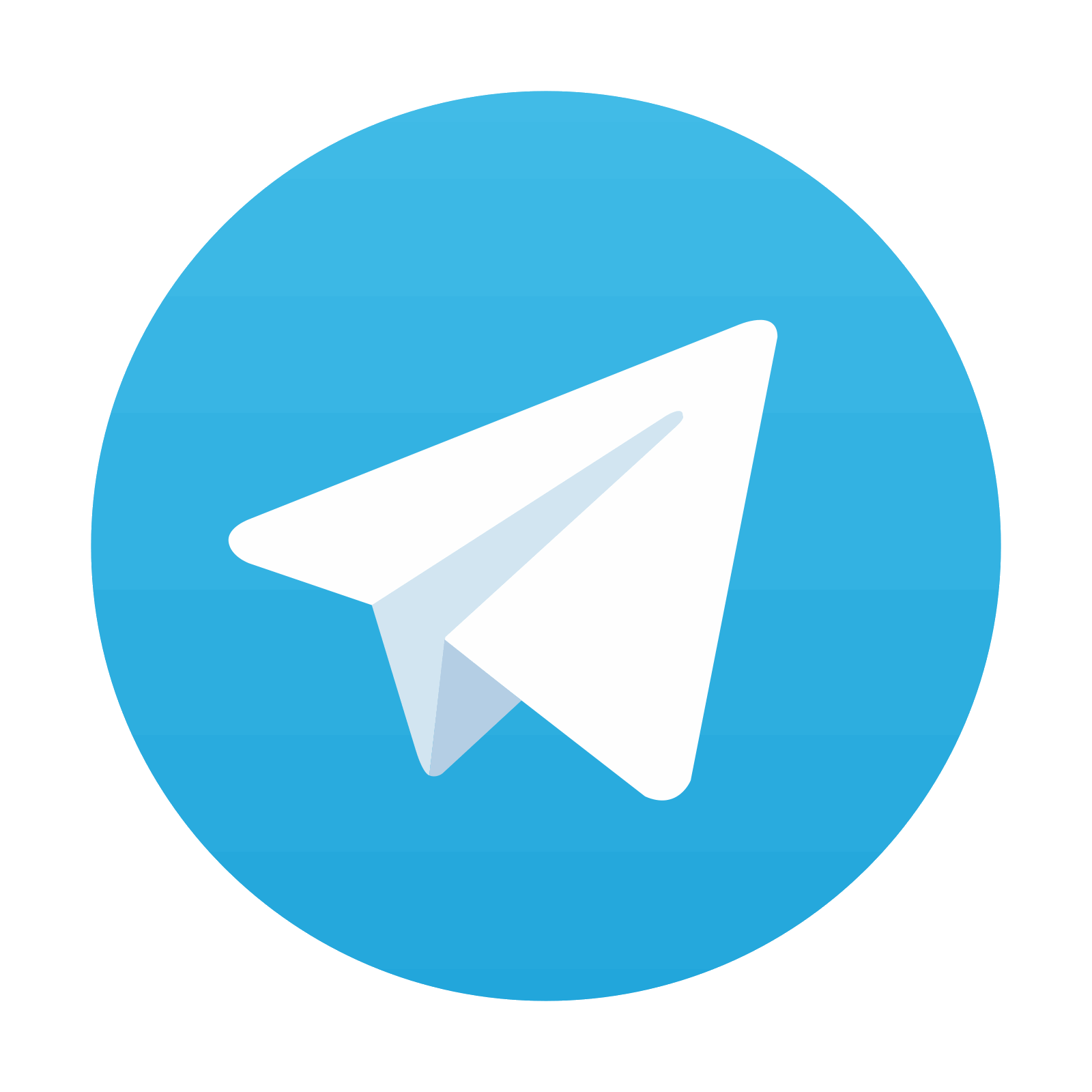
Stay updated, free articles. Join our Telegram channel
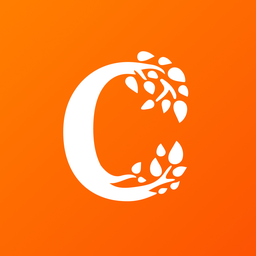
Full access? Get Clinical Tree
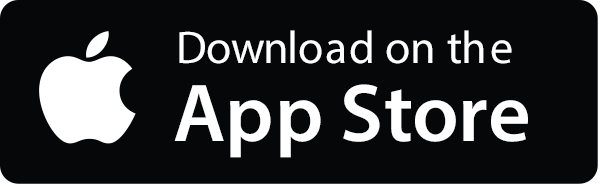
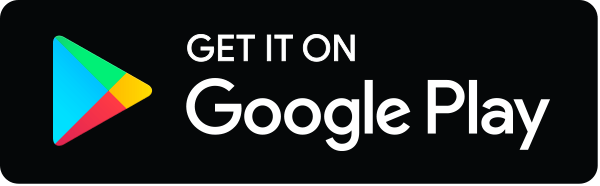