Nanotechnologies for Drug Delivery and Targeting Opportunities and Obstacles |
CONTENTS
5.1.1 Rationale for the Development of Parenteral Drug Delivery and Targeting Systems
5.1.2 Generalized Description of Parenteral Drug Delivery and Targeting Systems
5.2 Anatomical, Physiological, and Pathological Considerations
5.2.1 Enhanced Permeation and Retention Effect: Concept or Reality?
5.3 Passive and Active Targeting
5.4 Soluble Carriers for Targeted Drug Delivery
5.4.2 Soluble Polymeric Carriers
5.5 Particulate Carriers for Drug Targeting
5.5.1.1 Conventional Liposomes
5.5.1.2 Long-Circulating Liposomes
5.5.5 Polymeric Micro- and Nanoparticles
5.5.5.1 Poly(Alkyl Cyanoacrylates)
5.5.5.2 Poly(Lactic-co-Glycolic Acid)
5.5.5.3 Albumin and Other Protein Microspheres and Nanoparticles
5.5.7 Other Particulate Carrier Systems
5.6 Pharmaceutical Aspects of Carrier Systems
5.7 Obstacles to Successful Targeting
5.7.1.1 Particle Size and Particle Size Distribution
Routine parenteral administration by injection serves to deliver drugs to body tissues. The most important routes of injection of these sterile products are intramuscular (i.m.), intravenous (i.v.), and subcutaneous (s.c.). The process of developing conventional parenteral formulations involves the selection of appropriate vehicles (e.g., aqueous, oily, and emulsions) to achieve the desired bioavailability following injection, as described in Chapter 3. The present chapter focuses on advanced drug delivery and targeting systems (DDTS), in the nanometer range, administered via the parenteral route and serves to provide the reader with a basic understanding of the principle approaches to drug targeting.
5.1.1 RATIONALE FOR THE DEVELOPMENT OF PARENTERAL DRUG DELIVERY AND TARGETING SYSTEMS
As introduced in Chapter 2, there are many limitations associated with conventional drug therapy. An i.v.-administered drug is subject to a number of pharmacokinetic processes in vivo, which can decrease the drug’s therapeutic index, including the following:
• Distribution: Drugs administered i.v. distribute throughout the body and reach nontarget organs and tissues, resulting in drug wastage and consequently side effects and possibly toxicity.
• Metabolism: The drug may be rapidly metabolized in the liver or other organs.
• Excretion: The drug may be cleared rapidly from the body through the kidneys.
As a result of these processes, only a small fraction of the drug will reach the target tissue. Moreover, it may be cleared rapidly from this site and, therefore, not be available long enough to induce the desired effect. Reaching the target cell is often not the ultimate goal; in many cases, the drug has to enter the target cell to reach an intracellular target site. As discussed in Chapter 4, many drugs do not possess the required physicochemical properties to enter target cells; they may be too hydrophilic, too large, or labile or not transportable by the available active transport systems.
DDTS aim to overcome the limitations of conventional drugs and thus improve drug performance. An ideal DDTS should
• Specifically target the drug to target cells or target tissue
• Prevent the drug reaching nontarget organs, cells, or tissues
• Ensure minimal drug leakage during transit to target
• Protect the associated drug from metabolism
• Protect the associated drug from premature clearance
• Retain the drug at the target site for the desired period of time
• Facilitate transport of the drug into the cell
• Deliver the drug to the appropriate intracellular target site
• Be biocompatible, biodegradable, and nonantigenic
In certain situations, some of these requirements may be inappropriate. For example, the drug may work outside the cell; thus, cell penetration may not be necessary. In this chapter, there are also examples mentioned of passive targeting approaches (see Section 5.3.1), where the drug does not require to be specifically targeted to the cell or tissue.
The parenteral route of administration is associated with several major disadvantages. Parenteral administration is invasive and may require the intervention of trained medical professionals. Parenteral formulations often require refrigeration for storage. Strict regulations govern their use, which generally dictate that they are as simple as possible. The inclusion of excipients in the formulation is kept to an absolute minimum. Developing a DDTS requires an enormous amount of R&D investment in terms of cost, effort, and time, which can cause a significant delay in the development and marketing of a system and thus result in the final product being relatively expensive. Parenteral DDTS must, therefore, offer real therapeutic advantages to justify their use. Box 5.1 lists a number of pharmacokinetic considerations to decide if the use of DDTS is indicated for a particular drug.
BOX 5.1 PHARMACOKINETIC CONSIDERATIONS RELATED TO DRUG TARGETING
• Drugs with high total clearance are good candidates for targeted delivery.
• Carrier-mediated transport is suitable for response sites with a relatively small blood flow.
• The higher the rate of elimination of free drug from either central or response compartments, the greater the need for targeted drug delivery; this also implies a higher input rate of the drug carrier combination to maintain the therapeutic effect.
• For maximizing the targeting effect, the release of drug from the carrier should be restricted to the response compartment.
• The rate of release and quantity of drug released should be at the appropriate therapeutic level.
Drugs used in the treatment of diseases that are life threatening or that dramatically affect the quality of life of the patient are prime candidates for inclusion in a DDTS. Such drugs include those used in the treatment of cancer, as well as life-threatening microbial, viral, and fungal diseases. Chronic diseases such as arthritis can also be found on the priority list.
5.1.2 GENERALIZED DESCRIPTION OF PARENTERAL DRUG DELIVERY AND TARGETING SYSTEMS
The technology used for targeted drug delivery with carrier systems differs from the technology to achieve prolonged release profiles for a drug. If prolonged release of a drug via the parenteral route is required, s.c. or i.m. injection of a controlled release system is the first option to consider. The relevant technology is already available and validated for many years. Long-acting injections and implants are the subject of Chapter 6; some examples include
• The long-, medium-, and short-acting insulin formulations, prepared by crystal manipulation or physical complex formation
• Depot injections (aqueous suspensions, oily injections) of contraceptives and psychotropic drugs (such as fluphenazine esters)
• Polymeric implants, for example, Zoladex®, a biodegradable matrix implant of the luteinizing hormone-releasing hormone (LHRH) agonist goserelin, for s.c. administration
• Infusion pumps
In contrast, a DDTS generally comprises three functionally specific units, as listed in Table 5.1: the drug or therapeutic agent, the carrier system, and a “homing” ligand.
A “homing” ligand is a target-specific recognition moiety. For example, galactose receptors are present on liver parenchymal cells; thus, the inclusion of galactose residues on a drug carrier can allow the interaction of the carrier to the target cells, once the two are in close proximity. A number of different target-specific recognition moieties are available and discussed further in the succeeding texts. However, an important point to note here is that target-specific recognition moieties are not idealized “magic bullets,” capable of selectively directing the drug to the appropriate target and ignoring all other nontarget sites. Although the so-called homing ligand can increase the specificity of the drug for its target site, the process must rely on the (random) encounter of the homing ligand with its appropriate receptor during its circulation lifetime. This is a stochastic process, that is, one involving elements of chance rather than certainty; for example, particle escape from the circulation (extravasation) or a ligand–receptor interaction leading to cellular uptake. Box 5.2 lists the main relevant processes that have an element of uncertainty in terms of outcome.
TABLE 5.1
Components of Drug Delivery and Targeting Systems
Drug Delivery and Targeting Systems Component | Purpose |
The active moiety | To achieve the therapeutic effect |
The carrier system, which can be either soluble or particulate | To effect a favorable distribution of the drug To protect the drug from metabolism To protect the drug from early clearance |
A “homing” liganda | To specifically target the drug to the target cells or target tissue |
a Not necessary when “passive” targeting approaches are used. |
The carrier systems that are presently on the market or under development can be classified into two groups based on their size:
1. Soluble macromolecular carriers
2. Particulate carrier systems
This classification is sometimes rather arbitrary, as some soluble carriers are large enough to enter the colloidal size range. A useful distinction is that with macromolecular carrier systems, the drug is “covalently attached” to the carrier and has to be released through a chemical reaction. In contrast, with colloidal carriers, the drug is generally “physically associated” and does not need a chemical reaction to be released. Here, diffusion barriers in the carrier comprise the major barriers for premature release from the system. Stimulus-sensitive systems are also being devised to ensure that drug is only released once the systems have reached the target. Release in this case is instigated by an external source, such as heat or magnetic force, the subject of Chapter 14.
BOX 5.2 STOCHASTIC PROCESSES IN VECTOR-MEDIATED DRUG TARGETING
Definition: A stochastic process involves some random variable or variables, that is, it involves chance events or a probability, rather than a certainty, of an event occurring. Four examples are as follows:
1. Aggregation of nanoparticles under shear flow in the blood circulation or in static conditions, e.g., in cell culture. Aggregation can cause jamming of particles and would change the apparent size—biological action paradigm.
2. Extravasation. The escape from the circulation after i.v. administration.
3. Nanoparticle ligand–receptor interactions. Requiring both close approach (to within several nm) of particles and receptors and the correct orientation of vectors toward the receptor, both being random events.
4. Diffusion of vectors in tissues, which are complex environments involving iterative approaches to reach destinations.
Soluble carriers include antibodies and soluble synthetic polymers such as poly(hydroxypropyl methacrylate), poly(lysine), poly(aspartic acid), poly(vinylpyrrolidone), poly(N-vinyl-2-pyrrolidone-co-vinylamide), and poly(styrene-co-maleic acid anhydride).
Many particulate carriers have been designed for drug delivery and targeting purposes for i.v. administration. They usually share three characteristics:
1. Their size range: the minimum size is approximately 10 nm and the maximum size relevant for drug targeting is approximately 10–300 nm.
2. They are all biodegradable, but at different rates.
3. The drug is physically associated with the carrier, and in general, drug release kinetics are controlled by either diffusional transport or matrix degradation, or both.
Figure 5.1 shows a selection of some of the wide variety of both soluble and particulate DDTS that are currently under development or commercially available.
A full appreciation of the respective advantages and disadvantages of soluble and particulate carriers cannot be gained without first taking into account the relevant anatomical, physiological, and pathological considerations in vivo, described next.
5.2 ANATOMICAL, PHYSIOLOGICAL, AND PATHOLOGICAL CONSIDERATIONS
The body is highly compartmentalized and should not be considered as a large pool without internal barriers for transport. A schematic figure (Figure 5.2) illustrates the organs involved. The degree of body compartmentalization or, in other words, the ability of a macromolecule or particulate to move around, depends on its physicochemical properties, in particular
• Molecular weight/size
• Charge
• Surface hydrophobicity
• Surface attached ligands for interaction with surface receptors.
The smaller its size, the easier a drug carrier can passively move from one compartment to another. An important question is whether, and where, the carriers can pass through the endothelial lining of the blood circulation. Under physiological conditions, the situation exists as depicted in Figure 5.3. A continuous endothelium, as found in most parts of the body, comprises a continuous tube of endothelial cells, separated by intercellular clefts, i.e., narrow gaps between neighboring endothelial cells (Figure 5.3a). The cells are positioned on a continuous basal membrane. The exact characteristics of this barrier are still under investigation, but it is clear that particulate systems greater than 10 nm cannot pass through. Fenestrated capillaries, as found, for example, in the kidney and most endocrine glands, have many small pores (fenestrations) in the plasma membrane of the endothelial cells, ranging from 70 to 100 nm diameter (Figure 5.3b). Again, the subendothelial basement membrane is continuous. Sinusoidal capillaries (Figure 5.3c) are found in the liver, spleen, and bone marrow. The endothelial cells have large fenestrations, as well as large intercellular clefts, that allow the movement of molecules such as proteins through the capillary walls. The subendothelial basement membrane is either absent (as in the liver) or present as a fragmented interrupted structure (as in the spleen and bone marrow). Sinusoids in the liver contain phagocytic Kupffer cells, which remove bacteria and other particulates from the blood.
This anatomical information has important implications for the rational design of targeted carrier systems. If a therapeutic target is located outside the blood circulation and if normal anatomical conditions exist around the target site, a small-sized macromolecular carrier must be selected, in order to achieve sufficient “escaping tendency” from the blood circulation. Particulate carriers will generally fail to extravasate, simply because there is no possibility for endothelium penetration in normal circumstances.
In addition to the issue of endothelial permeability, the effect of macrophages in direct contact with the blood circulation (e.g., Kupffer cells in the liver) on the disposition of carrier systems must be considered. Unless precautions are taken, particulate carrier systems are readily phagocytized by these macrophages and tend to accumulate in these cells. Phagocytic uptake by the cells of the mononuclear phagocyte systems (MPS), also known as the reticuloendothelial system (RES), has been introduced in Chapter 4 (Section 4.3.3). The MPS constitutes an important part of the body’s immune system and comprises both fixed cells, such as macrophages in the liver (also known as Kupffer cells), spleen, lungs, bone marrow, and lymph nodes, and mobile cells such as blood monocytes and tissue macrophages. Its functions include
• The removal and destruction of bacteria
• The removal and metabolism of denatured proteins
• Antigen processing and presentation
• Storage of inert colloids
• Assisting in cellular toxicity
The cells of the MPS are always on the alert to phagocytize “foreign body–like material.” Thus, aside from being responsible for the removal of particulate antigens such as microbes, other foreign particulates, such as microspheres, liposomes, and other particulate carriers, are also susceptible to MPS clearance.
Clearance kinetics by the MPS are highly dependent on the physicochemical properties of the “foreign” particulate; on particle size, charge, and surface hydrophobicity; and perhaps, on shape. These properties are considered in turn.
Particle size: Particulates in the size range of 0.1–7 μm tend to be cleared by the MPS, localizing predominantly in the Kupffer cells of the liver.
Particle charge: For liposomes, it has been shown that negatively charged vesicles tend to be removed relatively rapidly from the circulation, whereas neutral vesicles tend to remain in the circulation for longer periods.
Surface hydrophobicity: Hydrophobic particles are immediately recognized as “foreign” and are generally rapidly covered by plasma proteins known to function as opsonins, which facilitate phagocytosis. The extent and pattern of opsonin adsorption depends highly on surface characteristics such as charge and hydrophilicity. Strategies to decrease MPS clearance, by increasing the hydrophilicity of the particle surface, are described in Section 5.3.1.
A further consideration is that in some “pathological” conditions, the endothelium exhibits modified characteristics. In general, the permeability is enhanced; the phenomenon is called the “enhanced permeability and retention” (EPR) effect, coined by Hiroshi Maeda, as will be discussed in Section 5.2.1. For example, the endothelial fenestrations in inflammation sites can be as large as 20 nm. In tumor tissue, even larger fenestrations can be found. However, in this case, the pattern is not uniform and depends on the tumor type and stage of development. Even within one tumor, highly permeable sites can be identified in close proximity to sites of low permeability. Also, necrotic tissue affects tumor permeability. Because of the EPR effect, particles in the colloidal size range can “theoretically” enter tumor tissue or sites of inflammation. This phenomenon can be exploited for drug delivery. However, while the EPR effect has been demonstrated in animals, there has been no clear evidence of the effect in human tumors. The EPR effect has been invoked in many publications as a means of access of carrier systems to such sites, but the evidence for the mechanism, or mechanisms, of accumulation is often not to be found. Extrapolation of animal data to humans is less than clear, as it is with other phenomena too. The status of this putative mechanism and some of these questions are discussed further for example in Sections 5.2.1, 5.5.1.1 and 5.5.1.2.
Shape: The development of asymmetric carbon nanotubes has given the opportunity to investigate the influence of shape on many processes. Most current vector systems are spherical or quasi-spherical, with different degrees of flexibility. But shape clearly affects the movement and behavior of the systems, for example, their transport through pores (see Figure 5.4) and their interaction with biomembranes. Shape can affect endocytosis: elongated particles have been found to be more readily endocytosed than spherical equivalents. Cytotoxicity is also influenced by shape, as asbestos has taught us. Carbon nanotubes are said to have a propensity for membrane interactions, but more work is required to elucidate all the shape-related factors in attachment and uptake.
5.2.1 ENHANCED PERMEATION AND RETENTION EFFECT: CONCEPT OR REALITY?
Much of the research carried out on vector-mediated targeting is performed in small animal models, usually rodents. In these animal models, a certain degree of accumulation of vectors has been observed, for example, at tumor sites, with both active and passive carrier systems, a feature habitually ascribed to the EPR effect. A certain accumulation indeed occurs in rodents, but this has rarely exceeded about 5% of the vector. Imaging techniques show this modest accumulation but unless the drug itself can be visualized, the data are optimistic, as the drug may be trapped within the carrier particles. In addition, the literature is difficult to interpret as it has been found that different imaging techniques can lead to different data. The EPR effect has been considered recently by a number of experts in a consensus paper (Prabhakar et al. 2013) that points out the heterogeneity of the EPR effect and the limited evidence for the effectiveness of the mechanism, which was originally proposed, as stated in this chapter, for (flexible) macromolecules rather than (rigid) nanoparticles.
Nanoparticle uptake is often determined by radiolabeling of the vector and not the drug. The only sensible approach is to measure the drug in the tumor and to distinguish active free drug, from the drug that is still bound to the carrier. One problem in assessing true targeting is that the capacity of a vector system for the drug is crucial, and hence, the same vector carrying different drugs might produce quite different results. Drug load can be high, as in many liposomes, but in some nanoparticles, it can be as low as 5% w/w. Should 10% of such a carrier reach the target, and the available drug is only 5% of the vector mass, the so-called drug targeting represents only 0.5% of the administered dose. Many studies suggest that maximal vector uptake is in the vicinity of 5%: arithmetic matters!
The rate of release and diffusion of drug from the carrier is clearly another issue in effective therapeutic action. Distribution of drug throughout tumor tissue is not, unsurprisingly, homogeneous since tumors are not themselves homogeneous. In tumor tissue extracellular matrix barriers, necrotic tissue and pressure differences may form barriers for access to parts of the diseased tissue in spite of pore formation in the endothelium. Generally speaking, the larger the carrier, the more its penetration is hindered. And drugs such as doxorubicin (although a small molecule) do not necessarily diffuse freely through tumor tissue, not only because of the complex diffusion pathways but also as they can bind to cellular and tissue components.
And then the following question has to be answered: how predictive are animal models for the therapeutic effects in the human patient? As seen in a few observations, the tumor cell lines used in the most “popular” animals (rodents) are fast growing (days, weeks). These tumor cell lines are rather homogeneous compared to human tumors; furthermore, the immune systems in rodents differs from man. Not only that, but adsorption of proteins onto the surface of nanoparticles in the blood varies with residence time in the circulation. Both the plasma proteins in rodents and the dynamics of circulation are different from those in man. Therefore, direct extrapolations from findings in animals to humans can only be made after careful validation studies. In some patients, indeed a degree of accumulation in the primary tumor and metastases has been demonstrated by imaging techniques, but the same imaging techniques showed no EPR effect in the majority of other patients.
5.3 PASSIVE AND ACTIVE TARGETING
Passive targeting exploits the “natural” (passive) distribution pattern of a drug carrier in vivo and no homing ligand is attached to the carrier. For example, as described earlier, particulate carriers tend to be phagocytized by cells of the MPS. Consequently, the major organs of accumulation are the liver and the spleen, both in terms of total uptake and uptake per gram of tissue. An abundance of MPS macrophages and a rich blood supply are the primary reasons for the preponderance of particles in these sites. After phagocytosis, the carrier and the associated drug are transported to lysosomes, and the drug is released upon disintegration of the carrier in this cellular compartment. This passive targeting to the MPS (and particularly to the liver) is advantageous in a number of situations, including
• The treatment of macrophage-associated microbial, viral, or bacterial diseases (e.g., leishmaniasis)
• The treatment of certain lysosomal enzyme deficiencies
• The immunopotentiation of vaccines (further information on the use of nanoparticles for vaccines is given in Chapter 17)
• The activation of macrophages, by loading the carrier system with macrophage-activating agents such as interferon γ, to fight infections or tumors
If the drug is not broken down by the lytic enzymes of the lysosomes, it may be released in its active form from the lysosomal compartment into the cytoplasm and may even escape from the phagocyte, causing a prolonged release systemic effect.
Technology is available to reduce the tendency of macrophages to rapidly phagocytize colloidal drug carrier complexes. The process of “steric stabilization” involves the coating of the delivery system with synthetic or biological materials, which make it energetically unfavorable for other macromolecules to approach the surfaces carrying these chains. A standard approach is to graft hydrophilic, flexible polyethylene glycol (PEG) chains to the surface of the particulate carrier (termed “PEGylation”). This highly hydrated PEG layer reduces the adsorption of opsonins from the plasma and consequently slows down phagocytosis. The net effect of PEG attachment is that macrophage/liver uptake of the particles is delayed or reduced, thus increasing the circulation time.
In order to avail of the EPR effect, two conditions should be satisfied:
1. The size of the drug carrier system should exceed the size of normal endothelial fenestrations to ensure that the carrier system only crosses inflamed/tumor endothelium; a certain size range is preferred as there is an upper limit to the endothelial fenestration dimensions under pathological conditions.
2. The circulation time in the blood compartment should be long enough to allow the carrier systems to “escape” from the circulation at the pathological site, though not all will.
As described earlier, the circulation time of a particulate carrier in the blood can be prolonged using “stealth” technology to enhance particle hydrophilicity. If the circulation time is sufficiently prolonged and the particle size does not exceed, say, 200 nm, then accumulation in tumor and inflammation sites can be observed. The goal today is to enhance accumulation in targets, which in tumors in man has rarely exceeded around 5% of the vector.
In active-targeting strategies, a specific ligand is attached to the carrier system, to improve delivery to a specific cell, tissue, or organ. Thus, delivery systems designed for active-targeting are usually composed of three parts: the carrier, the ligand, and the drug. Preferably, the ligand is covalently attached to the carrier, although successful targeting attempts of noncovalently attached ligand–carrier combinations have also been described.
Target sites for active-targeting strategies can differ widely. A list of cell-specific receptors and their corresponding ligands, expressed under physiological conditions, is presented in Table 5.2. Thus, for example, galactose can be used to target a drug carrier to parenchymal liver cells. Receptors are not always exposed directly to the vector, which might thus have to access the target and then interact. In the future, it is expected that the rapidly growing field of genomics will be used to identify specific receptors for targeting purposes, which might enhance the effectiveness of targeting.
Other receptors may become available under pathological conditions. Such receptors include
• Antigenic sites on pathogens (bacteria, viruses, parasites)
• Infected host cells expressing specific antigenic structures
• Tumor-associated antigens (TAAs) (i.e., antigenic structures specifically occurring at the surface of tumor cells)
• Substances such as fibrin in blood clots (i.e., potential ligands for targeting of fibrinolytics)
TABLE 5.2
Examples of Cell-Specific Ligands/Carriers In Vivo
Cell | Cell-Specific Ligands/Carriers |
Parenchymal liver cells | Galactose, polymeric IgA, cholesterol ester—VLDL, LDL |
Kupffer cells | Mannose/fucose, galactose (particles), (oxidized) LDL |
Liver endothelial cells | Mannose, acetylated LDL |
Leucocytes | Chemotactic peptide, complement C3b |
Abbreviations: VLDL, very-low-density lipoproteins; LDL, low-density lipoproteins. |
Sometimes it is necessary for the carrier-bound drug to reach all target cells to be clinically successful, as is the case with antitumor therapy. The so-called “bystander” effects can help to achieve fully effective therapy. Bystander effects occur when the targeted drug carrier reaches its target site, and released drug molecules also act on surrounding nontarget cells. In other cases, not all target cells have to be reached, as is the case, for example, for targeted gene delivery for the local production of a therapeutic protein. However, the cell nucleus is a target that is difficult to reach, as several barriers inside the cell have to be overcome (e.g., cytoplasmic membrane, endosomal wall, and the nuclear membrane).
Antibodies raised against a selected receptor are extensively used as homing ligands, as described in the succeeding texts. Modern molecular biotechnology permits the production of large amounts of tailor-made material. Other potential candidates are also emerging, in the cytokine and the growth hormone family and, finally, among the adhesion molecules that play a role in the homing of inflammatory cells to inflammation sites.
5.4 SOLUBLE CARRIERS FOR TARGETED DRUG DELIVERY
As described earlier, the major advantage of soluble carriers over particulate carriers is their greater ability to extravasate; also, being soluble, they can be taken into cells via the process of pinocytosis. Active-targeting strategies for soluble carriers include attaching rather simple ligands such as galactose, for targeting to liver parenchymal cells (see Table 5.2); alternatively, more complicated structures, such as antibodies, or antibody fragments (Fab or single-chain antibodies [SCA]) can be used as targeting ligands.
However, a number of disadvantages are also associated with the use of soluble carriers:
• Limited drug-loading capacity: a low ratio of drug to carrier limits the mass transport mediated by the drug carrier.
• The drug is covalently bound to the carrier: this can mask the active site of the drug and the conjugation reaction may damage a labile drug moiety.
• The carrier confers limited protection on the drug.
• The rate of cleavage of the drug from the carrier may not be optimal for activity.
The therapeutic antibodies that are on the market all come from the IgG family, with the IgG1 isotype being the most commonly used. Monoclonal antibodies (MAbs) belong to the largest molecules in our therapeutic arsenal with a molecular weight (MW) of 150,000 (1000 times the MW of paracetamol/acetaminophen). As shown in Figure 5.5, the structure consists of two heavy and two light chains. A sugar chain is connected to each heavy chain. The antigen-binding site of IgG molecules represents the homing part, which specifically interacts with the target (cells, pathogens, tissue). These antigen-binding sites are located at both tips of the Y-shaped molecules. The sites that are responsible for the pharmacological effects of IgG, such as complement activation and macrophage interaction, are located at the stem part of the Y. The rest of the molecule forms the connection between the homing ligand and the pharmacologically active sites, and also contributes to the long blood circulation characteristics of the IgG molecule, which has an elimination half-life much greater than 24 hours.
Humanized or human antibodies have replaced earlier generations of murine antibodies. There are four options in basic structure. The earliest generation was fully based on mouse hybridoma technology; then, the Fc part was mouse derived, with the Fab fragment comprising a human sequence (chimeric MAb), followed by humanized antibodies, where only the complementarity-determining region was mouse derived. Nowadays, fully human MAbs have entered the field. Mouse-derived antibodies can induce human antimouse antibodies (HAMA) in the patient. In multiple injection schemes, this HAMA reaction can cause neutralization of the homing capacity of the homing ligand, loss of activity, and anaphylactic reactions. Chimeric, humanized, and human antibodies induce HAMA (much) less frequently but, for instance, can still raise anti-idiotypic antibodies against the binding site structure. These anti-idiotypic antibodies can also interfere with the targeting performance.
MAbs have rather complicated names as shown in Figure 5.6. The WHO International Nonproprietary Name (INN) system provides the logic behind these names (see WHO 2008). Murine MAb names end with –momab, chimeric with –ximab, humanized with –zumab and, finally, fully human MAb with –mumab. The therapeutic indication is also referred to, e.g., –li– for an immunomodulating and –tu– for an antitumor effect. This helps to understand INN such as trastuzumab and infliximab.
The large size of MAbs (around 15 × 8 × 5 nm) and their hydrophilicity have some disadvantages. Penetration through endothelial and epithelial barriers and transport through extracellular spaces are slow. This leads to low uptake percentages at the target site if the target is located beyond the vascular endothelium (extravascular space). For instance, in cancer patients, the uptake of MAb in solid tumors is around 3% of the dose, which means that 97% ends up somewhere else in the body. Therefore, to facilitate (diffusional) transport, often the full antibody molecule (MW 150 kDa) is not utilized for target ligand (i.e., specific receptor) binding, but only the antigen-binding domain carrying the Fab (MW 50 kDa) fragment or even smaller fragments (SCA, MW 25 kDa).
In 1986, the first marketed MAb for therapeutic use was the mouse anti-CD3 antibody muromonab (OKT3), for the prevention of rejection of kidney transplants. Since then, a long list of MAb has been approved mainly for oncology, autoimmune diseases, and transplant rejection. In 2012, 5 out of 10 on the list of the globally most successful medicines (in terms of revenue) were MAbs, a clear indication of the fast-growing success of this family of medicines.
MAbs against TAAs have been developed to assist in tumor imaging. The MAb is conjugated with a diagnostic imaging agent (e.g., 111In). Commercial products include
• Satumomab pendetide (Oncoscint® CR/OV), for colorectal and ovarian adenocarcinomas
• Capromab pendetide (Prostascint®), for prostate cancer
• Arcitumomab (CeaScan®), for a number of carcinoembryonic antigen (CEA)-rich tumors
The therapeutic use potential of MAb as an “all-in-one” bioactive and targeting ligand molecule per se may be limited because of the limited immunological activity on the Fc part. Therefore, antibody–drug conjugates (ADC) have been developed. An example of an ADC is the MAb–drug conjugate, Adcetris® (brentuximab vedotin). This ADC is used for the treatment of Hodgkin lymphoma patients. Adcetris® consists of three components:
1. The chimeric IgG1 antibody cAC10, specific for human CD30 (exposed on lymphoma cells), which binds (random collision events, as well as access issues permitting, cf. stochastic paradigm, Box 5.2) to the tumor.
2. Monomethyl auristatin E (MMAE), a microtubule-disrupting agent (anticancer agent).
3. A protease-cleavable linker that covalently attaches MMAE to cAC10. It is stable outside the cell, and upon cell internalization, it is cleaved, releasing MMAE.
Bispecific antibodies are manufactured from two separate antibodies to create a molecule with two different binding sites. One binding site links the MAb to the target cell. The other site is chosen to bring T-lymphocytes or natural killer cells in close contact with the target site, in order to exert a pharmacological effect, for example, to kill the target cell. This approach is now in early stage clinical trials.
5.4.2 SOLUBLE POLYMERIC CARRIERS
Over the years, different soluble polymeric systems have been developed in attempts to enhance drug performance. Here again, the emphasis is on the improvement in drug disposition conferred by the carrier and ligand, as well as the protection offered by the system against premature inactivation. The strategy, as shown in Figure 5.7, involves the use of a soluble macromolecule, the molecular weight of which ensures access to the target tissue. The drug moiety can be bound via either a direct linkage or a short chain “spacer.” The spacer overcomes problems associated with the shielding of the drug moiety by the polymer backbone and allows cleavage of the drug from the polymer. The spacer allows greater exposure of the drug to the biological milieu thereby facilitating drug release. A targeting moiety, which can be either an integral part of the polymer backbone or covalently bound, may also be incorporated into the system.
FIGURE 5.7 Components of a soluble macromolecular site-specific delivery system.
A crucial feature of such carrier systems is their solubility, which enables them to be taken up into target cells by the process of pinocytosis (see Chapter 4, Section 4.3.3.1). The intact carrier enters the target cell through pinocytotic capture. Through an endosomal sorting step, the carrier reaches the lysosomes where it is exposed to the actions of a battery of degradative enzymes. The drug–carrier linkage is designed to be cleaved by these enzymes, liberating free, active drug that can leave the lysosome by passage through its membrane, reaching the cytoplasm and other parts of the cell. Intralysosomal release of the drug from the carrier can also be achieved by making the drug–carrier linkage acid labile, as the lysosomal interior has a pH of approximately 4.5–5.5.
Poly(N-(2-hydroxypropyl)methacrylamide) has been investigated as a soluble macromolecular carrier system, using doxorubicin as the active drug. The bulk of the conjugate consists of unmodified HPMA units, which comprise about 90% of the carrier; the remaining units are derivatized with doxorubicin (see also Chapter 1, Figure 1.10). A tetrapeptide spacer (sequence Gly-Phe-Leu-Gly) connecting doxorubicin to the HPMA units proved to be cleavable by lysosomal thiol proteinases. Enzymatic cleavage breaks the peptide bond between the terminal glycogen and the daunosamine ring, liberating free doxorubicin, which can diffuse to the cytoplasm and nucleus where it (presumably) exerts its action.
Targeting moieties can also be incorporated into this delivery system. Targeting systems that have been investigated include
• Galactose: for targeting to parenchymal liver cells
• Melanocyte-stimulating growth factor: for targeting to melanocytes
• MAbs: for targeting to tumors
Interestingly, the doxorubicin–polymer conjugate alone, without a target-specific ligand, showed an enhanced therapeutic index in animal models and considerable accumulation of the drug in tumor tissue. The EPR effect, as discussed earlier, is held responsible for this phenomenon. After optimizing conjugate performance in terms of doxorubicin “payload” and desired molecular weight range of the polymer backbone, clinical grade material is now available and clinical trials are in progress to evaluate the potential of this concept.
The cytotoxic neocarzinostatin (NCS) is a small protein (MW 12 kDa) associated with a low-molecular-weight chromophore. NCS is rapidly cleared by the kidney and its cytotoxicity is non–cell specific. To modify its disposition, two poly(styrene-co-maleic acid anhydride) copolymers (MW 1500) have been coupled to one molecule of NCS, to give styrene-maleic-anhydride-neocarzinostatin (SMANCS) systems (see also Chapter 1, Figure 1.11). It was the work with SMANCS that led Hiroshi Maeda to develop the concept of the EPR effect.
SMANCS has been shown to retain nearly all the in vitro activity of NCS, with much improved pharmacokinetic properties. Tumor uptake has been shown to increase in animal models. Clinical successes have been reported with SMANCS formulated in Lipiodol® (a lymphographic vehicle) after intra-arterial administration in patients with unresectable hepatocellular carcinomas. The fact that the drug is administered in this lipid vehicle perhaps complicates interpretation, but clinical trials, mainly in Japan, are still being conducted some decades after the compound’s first discovery.
One of the issues already touched upon is the need for optimization of drug loading and (too) early release from carrier systems. This can be an issue of polymer–drug compatibility in polymeric systems, although in liposomal systems interactions between drug and lipid are more conducive to high loadings (e.g., for doxorubicin liposomes discussed in Section 5.5.1). An alternative is to prepare drug–lipid conjugates, where the hydrophobic component is a compound such as squalene, which aggregates to form micelle-like structures with around 50% of drug content.
Coupling drugs to relatively low-molecular-weight insoluble or poorly soluble lipids, such as squalene—a natural lipid—has been proposed as a means of changing the biodistribution of the drug and to enhance activity. Using low-molecular-weight “carriers” means that the drug content is a significant element of the system. Gemcitabine, an anticancer nucleoside that is rapidly deactivated in vivo, displays an enhanced half-life and residence time when coupled to squalene. The gemcitabine–squalene compound (Figure 5.8) of which 40% is the drug payload, associates in aqueous solution because of its dual hydrophile–lipophile (surfactant) nature, forming aggregates of around 100–130 nm in diameter. These aggregates have a greater cytotoxic activity and accumulate to a higher extent in the liver and spleen than the drug itself. Other drugs have been coupled to squalene and clinical trials are in progress. Because of their structure, these squalene derivatives can also be readily incorporated into liposomes.
5.5 PARTICULATE CARRIERS FOR DRUG TARGETING
Advantages of particulate carriers include the following:
• The high drug loading that is possible with some systems.
• The drug does not have to be chemically attached to the carrier.
• A considerable degree of protection from degradation in vivo and control of drug release may be conferred on drug molecules encapsulated within the carrier.
The carrying capacity of a particulate is determined by the affinity of the drug for the carrier material: large proteins and peptides may have problems in mixing isotropically with polymeric core materials. This can lead to premature release of the drug.
However, a major limitation of these systems is their inability to cross continuous endothelial barriers (Figure 5.3) and leave the general circulation. In general, microparticulate carriers are phagocytized by the macrophages of the MPS, thereby rapidly localizing predominantly in the liver and spleen. However, sterically stabilized particulate carriers have extended circulation times and can remain in the blood; they either act as circulating drug reservoirs, or may slowly escape from the blood pool at pathological sites which exhibit increased vascular permeability.
Intravenously administered particles with dimensions exceeding a few μm (the diameter of the smallest capillaries) will be filtered by the first capillary bed they encounter, usually the lungs, leading to embolism. Intra-arterially administered particles with dimensions exceeding the diameter of the smallest capillaries (around 7 μm) will be trapped in the closest organ located upstream; for example, administration into the mesenteric artery leads to entrapment in the gut and into the renal artery leads to entrapment in the kidney. This approach is under investigation to improve the treatment of diseases in the liver. Arterial chemoembolization involves the use of drug-eluting microspheres, which combine the action of blocking the arterial blood supply to hepatic tumors, causing ischemia, and delivering drugs such as doxorubicin and irinotecan, which then have prolonged contact with the tumor.
Active-targeting strategies for particulate systems are similar to those discussed for soluble macromolecular systems (see Table 5.2 and Section 5.4.1 on antibodies).
Liposomes are vesicular structures based on one or more lipid bilayer(s) encapsulating an aqueous core (Figure 5.9). The lipid molecules are usually phospholipids, amphipathic moieties with a hydrophilic head group, and two hydrophobic chains (“tails”). Such moieties spontaneously orientate in water to give the most thermodynamically stable conformation, in which the hydrophilic head group faces out into the aqueous environment and the lipidic chains orientate inward avoiding the water phase; this gives rise to bilayer structures. In order to reduce exposure at the edges, the bilayers self-close into one or more concentric compartments around a central discrete aqueous phase. Dependent on the preparation protocol used, liposome diameters can vary between 20 nm and 20 μm, thus encompassing the nano- and microrange of diameters. In general, they can be multilamellar or unilamellar, i.e., a multitude of concentrically orientated bilayers surrounds the aqueous core or only one bilayer surrounds an aqueous core, respectively. However, other structures have also been described.
If multilamellar structures are formed, water is present in the core of the liposome and also entrapped between the bilayers. Depending on the physicochemical nature of the drug, it can either
• Be captured in the encapsulated aqueous phase (i.e., the aqueous core and the aqueous compartments between the bilayers) (hydrophilic drugs)
or
• Interact with the bilayer surface (e.g., through electrostatic interactions) or be taken up in the bilayer structure (lipophilic drugs)
Thus, liposomes can serve as carriers for both water-soluble and lipid-soluble drugs. The liposomal encapsulation of a wide variety of drugs, including antitumor and antimicrobial agents, chelating agents, peptides, proteins, and genetic material, have all been described.
Bilayer composition can be almost infinitely varied by choice of the constituent lipids. Phosphatidylcholine, a neutral phospholipid, has emerged as the major lipid component used in the preparation of pharmaceutical liposomes. Phosphatidylglycerol and phosphatidylethanolamine are also widely used. Liposomal bilayers may also accommodate sterols, glycolipids, organic acids and bases, hydrophilic polymers, antibodies, and other agents, depending on the type of vesicle required.
The rigidity and permeability of the bilayer strongly depend on the type and quality of lipids used. The alkyl-chain length and degree of unsaturation play a major role. For example, a C18 saturated alkyl chain produces rigid bilayers with low permeability at room temperature. The presence of cholesterol also tends to rigidify the bilayers. Such systems are more stable and can retain the entrapped drug for relatively longer periods, whereas more “fluid” bilayer systems can be prepared if a more rapid release is required.
Liposomes can be classified on the basis of their composition and in vivo applications:
• Conventional liposomes, which are neutral or negatively charged, are generally used for passive targeting to the cells of the MPS.
• Sterically stabilized (“stealth”) liposomes, which carry hydrophilic coatings, are used to obtain prolonged circulation times.
• Immunoliposomes (“antibody targeted”), which can be either conventional or sterically stabilized, are used for active-targeting purposes.
• Cationic liposomes, which are positively charged, are used for the delivery of genetic material.
As phospholipid bilayers form spontaneously when water is added, the important challenge in liposome preparation is not the assembly of simple bilayers (which happens automatically), but in causing the bilayers to form stable vesicles of the desired size, structure, and physicochemical properties, with a high drug encapsulation efficiency. There are many different approaches to the preparation of liposomes; however, what they all have in common is that they are based on the hydration of lipids.
Liposomes represent highly versatile drug carriers, offering almost infinite possibilities to alter structural and physicochemical characteristics. This feature of versatility enables the formulation scientist to modify liposomal behavior in vivo and to tailor liposomal formulations to specific therapeutic needs. It took decades to develop the liposome carrier concept to a pharmaceutical product level, but a number of commercial preparations are now available in important disease areas and many more formulations are currently undergoing clinical trials. Examples of the different applications and commercial products of various types of liposomal systems are given in the following texts.
5.5.1.1 Conventional Liposomes
These can be defined as liposomes that are typically composed of only phospholipids (neutral and/or negatively charged) and/or cholesterol. Most of the early work on liposomes as a drug carrier system employed this liposomal type. These systems are rapidly taken up by the phagocytic cells of the MPS, localizing predominantly in the liver and spleen, and are therefore used when targeting to the MPS is the therapeutic goal. Conventional liposomes have also been used for antigen delivery and a liposomal hepatitis A vaccine (Epaxal®) has received marketing approval in Switzerland.
A commercial product based on conventional liposomes has been introduced for the parenteral delivery of the antifungal drug, amphotericin B, which is associated with a dose-limiting nephrotoxicity in conventional formulations. AmBisome®, a liposomal formulation of amphotericin B, comprises small unilamellar vesicles with diameters between 50 and 100 nm. Two other lipid-based formulations of amphotericin B are used in the clinic:
• Abelcet® consists of ribbonlike structures having a diameter in the 2–5 μm range.
• Amphocil® comprises a colloidal dispersion of disk-shaped particles with a diameter of 122 nm and a thickness of 4 nm.
In spite of the large differences in structural features (a further example of “liposomal” versatility), all formulations have been shown to greatly reduce the kidney toxicity of amphotericin B, allowing higher doses to be given and thereby improving clinical efficacy.
5.5.1.2 Long-Circulating Liposomes
At present, the most popular way to produce long-circulating liposomes is to covalently attach the hydrophilic polymer, PEG, to the liposome bilayers. As discussed in Section 5.3.1, the highly hydrated PEG groups create a steric barrier against interactions with molecular and cellular components in the biological environment. Figure 5.10 shows how “PEGylation” of liposomes can extend their blood circulation profile.
Long-circulating liposomes can enhance their chances of extravasation and thus accumulate at sites where pathological reactions occur. For example, the commercial product Doxil® (marketed as Caelyx® in Europe) consists of small-sized PEGylated liposomes, encapsulating the cytostatic doxorubicin. The resulting long-circulation times and small size of the vesicles facilitate their accumulation in tumor tissue
DaunoXome® liposomes are also long-circulating liposomes, in this case encapsulating the cytostatic daunorubicin. Although a nonstealth system, long-circulation times are attained by using a particularly rigid bilayer composition, in combination with a relatively small liposome size. The encapsulation of these anthracycline cytostatics in liposomes affects a modified biodistribution of the drug; the drug is distributed away from the heart, where in free form it can exert considerable toxic effects, and is preferentially taken up by solid tumor tissue.
Immunoliposomes have specific antibodies or antibody fragments on their surface to enhance target site binding. The primary focus of their use has been in the targeted delivery of anticancer agents.
Long-circulating immunoliposomes can also be prepared. The antibody can be coupled directly to the liposomal surface; however, in this case, the PEG chains may provide steric hindrance to antigen binding. Alternatively, a bifunctional PEG linker can be used, to couple liposomes to one end of PEG chains and antibodies to the other end of these chains (Figure 5.9). Steric hindrance is not a problem in the latter approach.
Cationic liposomes demonstrate considerable potential for improving the delivery of genetic material. The cationic lipid components of the liposomes interact with, and neutralize, negatively charged DNA, thereby condensing the DNA into a more compact structure. Depending on the preparation method used, the complex may not be a simple aggregate, but an intricate structure in which the condensed DNA is surrounded by a lipid bilayer. These systems are discussed further in Chapter 16.
An alternative to phospholipid-based liposomes can be found in niosomes (nonionic surfactant vesicles) developed by L’Oreal for cosmetic use. These systems, which can be uni- or multilamellar, are based on several different families of synthetic, nonionic low hydrophilic-lipophilic balance (HLB) amphipathic molecules, such as sorbitan monolaurate and others of this series, or alkyl glucosides, all formed with cholesterol. While there is considerable experimental experience with niosomes as parenteral delivery systems, there are no clear advantages over liposomal systems: although composed of nonionic surfactants/cholesterol mixtures rather than phospholipid/cholesterol mixtures and thus having a potential for greater variation in their composition, they turn out to have very similar physical and biological properties.
As described in Chapter 3 (Section 3.8), when low MW amphipathic (surfactant) molecules with distinct hydrophilic and hydrophobic sections are dispersed in water, spherical micelles in the nanometer size range are formed above a certain concentration, the critical micelle concentration (CMC). These micelles can solubilize drug molecules in their hydrophobic core, a useful formulation technique that has been used for the preparation of injections of paclitaxel, for example. There is a constant exchange between the surfactant monomers and their micelles. The more hydrophobic the surfactant, the lower the CMC. Nonionic surfactant systems generally have very low CMCs so that the micellar phase can exist even after dilution. However, micelles used as carriers for drug-targeting purposes must be stable in the blood circulation and should not disintegrate upon contact with blood components so that the drug load can be kept on board. In spite of the low CMCs of surfactant molecules, they are fragile. Using polymeric amphipathic materials to form the so-called polymeric micelles allows the formation of more stable systems suitable for targeting. The rate of exchange between the micellar and monomeric forms is slow because of the strong interaction forces between the molecules in the polymeric micelle.
There are several types of polymeric surfactant. Micellar systems based on amphipathic block copolymers have been explored as i.v.-administered drug carrier systems. These block copolymers can be, for example, composed of a hydrophilic PEG block (A) and a hydrophobic block (B) based on poly(aspartic acid) or poly(β-benzyl-L-aspartate). These form micelles in aqueous solution with spherical core/shell structures having diameters around 20–40 nm (see also Chapter 1, Figure 1.14). The hydrophobic core of these micelles can be loaded with lipophilic drugs such as doxorubicin. After i.v. administration, the micelles may accumulate at tumor sites. Some of the characteristics of these micellar systems are listed in Box 5.3. A subset of polymeric micelles is formed by lipid-core micelles, which comprise systems formed from conjugates of soluble copolymers with lipids. One example is the PEG–phosphatidylethanolamine conjugate.
BOX 5.3 POLYMERIC MICELLES AS DRUG CARRIERS
• Critical micelle concentration of the amphipathic copolymers is low; interaction between polymer units is strong, so that blood components cannot disrupt the aggregates.
• Molecular weight of the polymeric unit is small enough to allow clearance through glomerular filtration.
• Diameter is large enough to prevent penetration through intact endothelium.
• Diameter can be chosen in the range where the EPR effect is observed (<200 nm).
• Release kinetics of the drug depend on the selected polymer structure (hydrophilicity/hydrophobicity balance).
• Drug is in the hydrophobic core of the micelle and is protected from exposure to aqueous degradation processes.
• They have been shown to have a high drug–carrying capacity (“payload”).
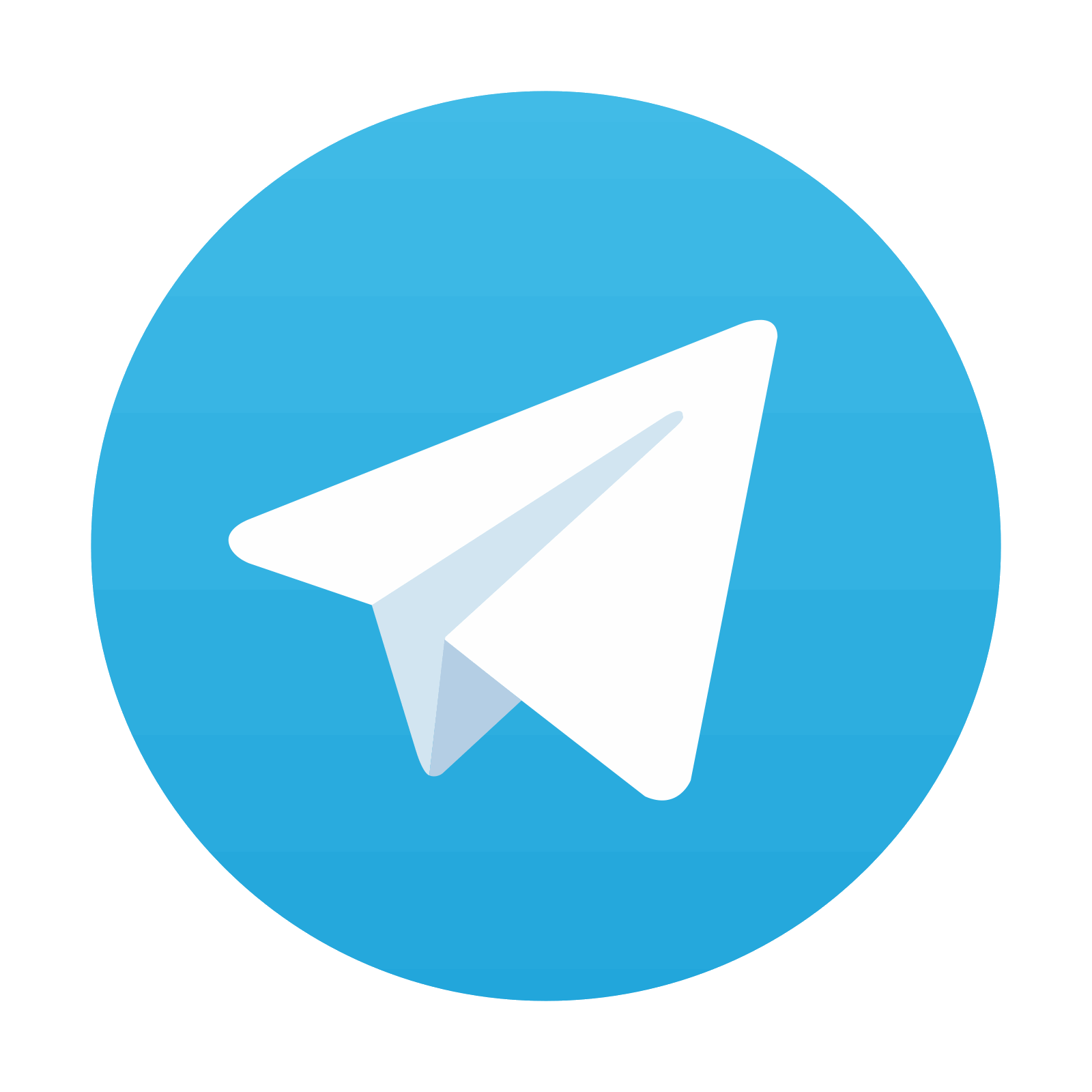
Stay updated, free articles. Join our Telegram channel
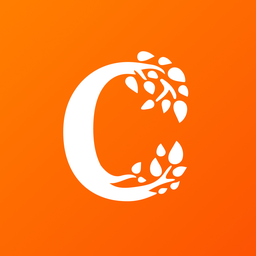
Full access? Get Clinical Tree
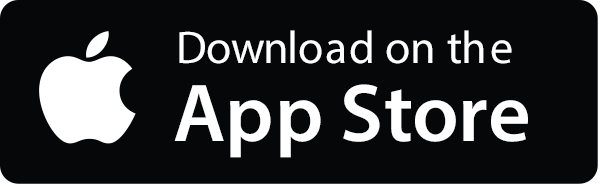
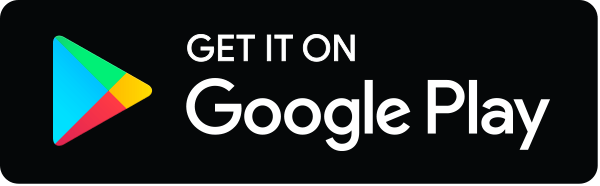