Metabolic Consequences of Starvation1
L. John Hoffer
1Abbreviations: ADP, adenosine diphosphate; BMI, body mass index; CED, chronic energy deficiency; FFM, fat-free mass; IGF, insulinlike growth factor; N, nitrogen; NPRQ, nonprotein respiratory quotient; REE, resting energy expendi SIRS, systemic inflammatory response syndrome; T3, triiodothy T4, thyroxine.
Starvation is the physical condition brought about by inadequate consumption, absorption, or retention of protein or dietary energy. The disease that eventually results from persistent starvation is protein-energy malnutrition. This chapter explains the physiology of starvation, which occurs both as a disease and in a nonpathologic form during therapeutic weight reduction. Pathologic starvation is usually caused by a general reduction of food intake; hence, it is commonly complicated by deficiencies of micronutrients as well as macronutrients (1, 2).
The physiology of starvation is central to human nutrition and many aspects of metabolism and medicine. Additional chapters in this edition deal with the clinical aspects of protein-energy malnutrition. This chapter explains the metabolic features of protein and energy insufficiency in humans, with the aim of establishing links between topics in physiology and clinical nutrition that are covered in other chapters in the text, including, among others, protein and energy metabolism, body composition, and nutritional assessment.
DEFINITIONS
Many terms have been used to describe starvation. In this chapter, starvation refers to states of negative protein or energy balance and their physiologic effects. A fast or total fast is a unique form of starvation in which all food energy is excluded. Terms such as starvation, inanition, emaciation, wasting, and cachexia have been used in the past interchangeably to describe the malnourished condition of famine victims, underfed prisoners, and people with chronic disease and important weight loss. In more recent years, cachexia has come to be used to refer specifically to the protein depletion induced by persistent, low-grade systemic inflammation or metabolic stress (3, 4, 5, 6). Advancing old age is associated with loss of muscle mass and function, termed sarcopenia (4, 5, 6). Although potentially modifiable by diet and lifestyle, sarcopenia is not considered as a form of starvation in this chapter.
Starvation takes different forms. The cardinal feature of prolonged fasting is ketosis (7). Contrary to what has been claimed sometimes, ketosis is neither sensitive nor specific as an indicator of starvation. Moreover, mild ketonuria may be present in healthy lean young adults after the overnight fast and people subsisting on a fully adequate but carbohydrate-restricted diet. Ketosis is prevented or abolished by carbohydrate intakes as low as 100 g/day (8); hence, it is absent in most starving people.
PROLONGED FASTING
Carbohydrate Metabolism
A description of fasting metabolism best proceeds from the last meal before the fast begins. Characteristic of the
fed state are increased blood concentrations of glucose, lipids, amino acids, and their metabolites. Carbohydrate and amino acid ingestion stimulates insulin secretion, which regulates their disposition within the tissues by stimulating glycogen, triglyceride, and protein synthesis while simultaneously inhibiting glycogenolysis, lipolysis, and proteolysis. Glucagon levels are unaltered or decreased after carbohydrate consumption, whereas protein consumption stimulates both glucagon and insulin secretion (7). Glucagon serves to stimulate liver glycogen breakdown and increase hepatic glucose output, thereby sustaining an adequate blood glucose concentration even as insulin stimulates glucose and amino acid uptake by peripheral tissues.
fed state are increased blood concentrations of glucose, lipids, amino acids, and their metabolites. Carbohydrate and amino acid ingestion stimulates insulin secretion, which regulates their disposition within the tissues by stimulating glycogen, triglyceride, and protein synthesis while simultaneously inhibiting glycogenolysis, lipolysis, and proteolysis. Glucagon levels are unaltered or decreased after carbohydrate consumption, whereas protein consumption stimulates both glucagon and insulin secretion (7). Glucagon serves to stimulate liver glycogen breakdown and increase hepatic glucose output, thereby sustaining an adequate blood glucose concentration even as insulin stimulates glucose and amino acid uptake by peripheral tissues.
The fed state ends after the last nutrient has been absorbed and the transition to endogenous fuel consumption gets underway. The condition that exists after the overnight fast is convenient for study and is termed the basal or postabsorptive state. This state is characterized by the release, interorgan transfer, and oxidation of fatty acids and the net release of glucose from liver glycogen and amino acids from muscle; all these processes are activated by the relatively low circulating insulin concentration that prevails in this situation. The body’s predominant postabsorptive fuel is fat. Thus, as indicated by the typical nonprotein respiratory quotient (NPRQ) of 0.8, fat oxidation typically accounts for two thirds of postabsorptive resting energy expenditure (REE) (9).
Under postabsorptive conditions, glucose enters the circulation and disappears into the tissues at a rate of 8 to 10 g/hour, replacing the body’s extracellular free glucose pool of approximately 16 g every 2 hours (10). Glucose is normally the only fuel available to the brain. Any lowering of the blood (and cerebrospinal fluid) glucose concentration lower than a critical level promptly impairs consciousness, and if prolonged, can lead to neuron death. Given the brain’s fixed and high metabolic requirement— approximately half the body’s total glucose production rate—there is no room for error or delay in the delivery of glucose from the liver into the systemic circulation. The blood glucose concentration of healthy individuals is closely regulated by several physiologic control systems, chief of which are the insulin and glucagon systems.
In the period that follows metabolic disposition of a meal, continual extraction of circulating glucose by the tissues progressively lowers the blood glucose concentration. Insulin levels fall in concert, thereby automatically slowing the rate of glucose transport into muscle and fat cells, while stimulating hepatic glycogenolysis and inhibiting hepatic glycogen synthesis to assure the continued release of adequate amounts of glucose from the liver into the bloodstream.
Hepatic gluconeogenesis (the synthesis of glucose from lactate, amino acids, and glycerol) is a continuous process, even in the fed state (11). Early in the postabsorptive period, approximately half the glucose appearing in the circulation originates from gluconeogenesis and the other half from hepatic glycogenolysis (10, 12). The precise relative contributions of gluconeogenesis and glycogenolysis to the circulating glucose pool depend on the carbohydrate and protein content of the preceding diet, for these respectively determine the size of the liver’s glycogen store and the rate at which glucogenic amino acids are reaching the liver to serve as a substrate for gluconeogenesis (13). As the fast is prolonged, glucose molecules derived from gluconeogenesis increasingly enter the circulation immediately rather than being sequestered in glycogen, and the liver gradually releases its entire glycogen store into the circulation. The kidneys are also gluconeogenic organs. Their fractional contribution to the circulating glucose pool increases as the liver’s glycogen store is expended and total hepatic glucose output decreases (14).
A fast longer than 12 to 24 hours reduces insulin levels yet further, causing a substantial mobilization of free fatty acids and glycerol from adipose tissue and amino acids from muscle (15). Delivery of these molecules to the liver provides energy and the substrate for protein synthesis and gluconeogenesis. Plasma glucagon concentrations remain constant or increase, thus lowering the insulin-glucagon ratio to activate the liver to oxidize the increased amounts of fatty acids now being delivered to it. Once activated in this way, the liver’s fatty acid oxidation rate is determined by the rate at which fatty acids are delivered to it (16). The rate of glucose conversion to coenzyme A, the entry substrate in the Krebs cycle, decreases importantly as the rate of fatty acid conversion to acetyl coenzyme A increases. Some of the acetyl coenzyme A produced from fatty acid oxidation is completely oxidized to carbon dioxide via the intrahepatic Krebs cycle, thereby serving as the liver’s predominant energy source (17), but most of it is oxidized only as far as the four-carbon molecule, acetoacetic acid, which spontaneously interconverts with its oxidoreduction partner, β-hydroxybutyric acid, and, to a lesser extent, is irreversibly decarboxylated to acetone. These three molecules are collectively termed the ketone bodies. Under prolonged fasting conditions, the liver acts as a factory that absorbs fatty acids delivered to it from adipose tissue, converts their carbon into ketone bodies, and exports them into the general circulation.
A fast longer than 2 or 3 days completely exhausts the liver’s approximately 80-g glycogen reserve (12, 18) and approximately half the glycogen in muscle (18, 19). The liver’s gluconeogenic rate neither increases or decreases, and therefore accounts for an increasing fraction of its total glucose output rate (14), which decreases by 40% to 50% within the first few days of fasting (12, 20). Muscle cells do not export glucose, so their residual glycogen plays no role in steady-state whole body carbohydrate economy. Consequently, once the liver’s glycogen store is completely exhausted, all the glucose that is oxidized in the body must be synthesized from three precursors: (1) the glucogenic amino acids; (2) glycerol released because of lipolysis;
and (3) lactate and pyruvate, molecules that, being the products of glycolysis, merely represent recycled glucose (21). The oxidation rate of preformed carbohydrate falls to zero, and as proof of this, the NPRQ falls to 0.7 (9).
and (3) lactate and pyruvate, molecules that, being the products of glycolysis, merely represent recycled glucose (21). The oxidation rate of preformed carbohydrate falls to zero, and as proof of this, the NPRQ falls to 0.7 (9).
Despite the marked reduction in hepatic glucose release, serum glucose concentrations decrease only moderately during fasting, because tissue glucose uptake and metabolism also decrease. Only part of this reduction in glucose metabolism is caused by reduced terminal glucose oxidation in muscle and adipose tissue, and none of it caused by a slower rate of lactate and pyruvate reconversion to glucose (the Cori cycle). An important reason for reduced tissue glucose use early in fasting, and the main reason for it in prolonged fasting, is a progressive switch by neural tissues to the use of ketone bodies as their energy supply (17, 22, 23). This phenomenon was elegantly demonstrated in a study of human subjects on a short-term fast in whom a combination of positron emission tomography and arterial-internal jugular vein sampling was used to measure glucose metabolism and β-hydroxybutyrate consumption. After 3.5 days of fasting, glucose consumption by the brain decreased by 25% and ketone body extraction increased correspondingly (24).
Ketosis
Ketosis (the presence of an abnormally high blood ketone body concentration) is the cardinal sign of prolonged fasting. Under nonfasting conditions acetoacetate oxidation furnishes only 2% to 3% of the body’s total energy requirement (17), and blood ketone body concentrations are almost immeasurably low (25). Starvation ketosis is arbitrarily defined as being present when the blood acetoacetate concentration rises to 1.0 mmol/L and β-hydroxybutyrate to 2 to 3 mmol/L, as typically occurs by day 2 or 3 of fasting (17). Ketone bodies are usually absent from overnight fasting urine, but mild ketonuria is not abnormal in thin healthy persons and indicates a physiologically low basal insulin state (26, 27). After their release into the blood, acetoacetic acid and β-hydroxybutyric acid dissociate to form water-soluble anions. Some acetoacetic acid is decarboxylated to acetone, and by day 3 or 4 days of fasting, its characteristic sweet odor is detectable in the breath.
Two factors determine the liver’s rate of ketone body synthesis. The first is the liver’s maximum capacity for fatty acid β-oxidation when fully activated by a low-insulin state. This rate depends both on the mass of perfused metabolically active liver tissue and the rate at which adenosine diphosphate (ADP) becomes available from adenosine triphosphate (ATP) hydrolysis, which, in turn, depends on the liver’s total energy expenditure rate (28). The second factor is the rate of adipose tissue triglyceride lipolysis, which determines the rate at which free fatty acids reach the liver.
The rate of ketogenesis is maximal as early as day 3 of fasting, but the blood ketone body concentration continues to rise over the ensuing days and weeks. There are two explanations for this phenomenon. First, muscle decreases its rate of ketone body oxidation, shifting to fatty acids as its preferred fuel. Second, the renal tubules reabsorb ketone bodies with greater efficiency. After the first 4 to 7 days of a total fast, ketone body oxidation accounts for 30% to 40% of the body’s total energy use. By week 3, a steady-state circulating ketone body concentration has been attained that is approximately twice the concentration that existed after the first 3 to 5 days. Because the brain uses ketone bodies in proportion to their delivery to it, brain ketone body oxidation steadily increases over this period as glucose oxidation decreases. After 3 to 5 weeks of fasting, brain glucose uptake is globally reduced by approximately 50% (17, 22). Moreover, by this time only 60% of the glucose taken up by the brain is fully oxidized to carbon dioxide and water, the rest being metabolized only as far as pyruvate and lactate, which return to the liver as gluconeogenic substrates (22). The combination of reduced terminal oxidation and increased local Cori cycling reduces the brain’s rate of irreversible glucose oxidation by 75%, with an equivalent reduction in the body’s requirement for gluconeogenesis from amino acids and glycerol.
Ketogenesis appears to be partly restrained by means of a negative feedback system which uses the circulating ketone body concentration as its sensor. Ketone bodies were long known to reduce lipolysis; but the mechanism for this effect was revealed more recently with the discovery of a G-protein-coupled receptor for niacin, a vitamin that, when administered in gram doses, potently inhibits lipolysis. The natural ligand for the niacin receptor has been identified as β-hydroxybutyrate (29). The physiologic mechanism that shifts muscle’s fuel preference from ketone bodies to fatty acids, after approximately 2 weeks of fasting, remains unexplained (10). Perhaps there is a role for the niacin receptor in this process.
Biologic Significance of Ketosis
Mention of ketosis or ketoacidosis (ketosis severe enough to lower the serum bicarbonate concentration but still within its normal buffering capacity) brings diabetes mellitus to mind. In the most severe form of this disease, destruction of the pancreatic β cells results in nearly total insulin deficiency. The result is mobilization of fatty acids and a priming of the liver for ketone body production and gluconeogenesis, as occurs in simple fasting (16, 30). When carbohydrate is ingested without coordinated insulin secretion, little of the glucose appearing in the circulation is taken up by muscle and adipose tissue. The blood glucose concentration rises to high levels, greatly exceeding the renal threshold for glucose reabsorption. The result is glycosuria and an osmotic diuresis that depletes the body of water and extracellular fluid. In prolonged fasting by nondiabetic persons, ketone body levels seldom rise higher than 6 to 8 mmol/L, but they rise much higher in diabetic ketoacidosis, imposing an acid load too
great for the body’s buffering system to absorb and causing a dangerous fall in pH. This condition is known as ketoacidemia.
great for the body’s buffering system to absorb and causing a dangerous fall in pH. This condition is known as ketoacidemia.
Why is the ketosis of simple fasting mild and clinically benign, whereas the ketoacidosis of diabetes commonly escalates into life-threatening ketoacidemia? Some understanding of the pathogenesis of severe ketoacidemia is provided by a consideration of the uncommon but welldocumented syndrome called nondiabetic ketoacidosis. This life-threatening disease typically occurs when protracted vomiting and volume depletion follows an alcoholic drinking binge during which there has been no food consumption. The ketoacidemia that develops under these conditions may be as severe as diabetic ketoacidosis, even though the blood glucose concentration remains close to normal (31, 32). Nondiabetic ketoacidosis also occurs rarely in pregnancy. As with alcoholic nondiabetic ketoacidosis, gestational nondiabetic ketoacidosis occurs in a setting of fasting, physiologic severe hypoinsulinemia, and volume depletion or metabolic stress (33).
Two features distinguish severe ketoacidosis from the benign ketoacidosis of fasting: volume depletion and hypermetabolism. Volume depletion worsens existing ketoacidosis (and worsens hyperglycemia) in various ways: by reducing blood flow to the kidneys and brain, reducing brain and renal ketone body oxidation, and greatly reducing urinary ketone body excretion (32). Normal fasting is a hypometabolic phenomenon, whereas uncontrolled diabetes and hypermetabolic volume depletion are characterized by hyperglucagonemia and increased norepinephrine secretion. These hormones increase free fatty acid delivery to the liver (34, 35) and potentially increase its rate of energy consumption, thus making more ADP available and boosting its ketogenic capacity (28) under conditions in which volume depletion has reduced the glomerular filtration rate and hence reduced renal tubular sodium reabsorption and correspondingly reduced the kidneys’ energy consumption and ketone body oxidation rate (32). The net effect is a large increase in circulating ketone bodies. During simple prolonged fasting, any stress-induced increase in the blood glucose concentration stimulates endogenous insulin release, which acts to reduce hepatic glucose release, restrain lipolysis, and inhibit ketogenesis (25). In severely volume-depleted states, this does not always happen, presumably because hyperadrenergic states inhibit insulin secretion (32). The notion that severe ketoacidosis arises in a setting of hypermetabolism was already appreciated in the preinsulin era. Before 1922, the only treatment that extended the life of insulin-dependent diabetic patients was a diet low in carbohydrate, which prevented hyperglycemia, glycosuria, and volume depletion, and a diet low in total energy, which reduced the patient’s metabolic rate and hence the liver’s ketogenic rate (36).
It is commonly repeated in the popular media that ketone bodies are toxic because the ketonuria associated with dietary carbohydrate restriction “damages the kidneys.” This claim lacks a scientific basis. Perhaps the notion that ketone bodies are toxic arose because they partially inhibit the urinary excretion of urate (10), and this effect— especially in the setting of extracellular volume depletion, which increases renal tubular urate reabsorption (37, 38)— increases the serum uric acid concentration. Thus an attack of gout may occur in susceptible persons during total fasting or severe carbohydrate restriction. The possibility has been raised that hyperketonemia during pregnancy could adversely affect the fetal brain (27) or predispose to congenital malformations (39). It is certainly true that the rapid glucose use characteristic of late pregnancy predisposes to fasting hypoglycemia, hypoinsulinemia, mild ketosis, and ketonuria (40), and that ketone bodies are used as fuel by fetal tissues. However, no plausible mechanism has been advanced to explain why any of these effects would be harmful, and the clinical evidence linking ketonuria with adverse fetal outcomes is unconvincing (41). Nevertheless, it remains a common practice to counsel pregnant women to avoid periods of prolonged fasting (27).
In summary, prolonged fasting is characterized by a low blood glucose concentration, physiologic hypoinsulinemia, and moderate ketosis, whereas uncontrolled insulindependent diabetes is characterized by hyperglycemia, volume depletion, hypermetabolism, and severe ketosis, all of which are the direct or indirect result of severe insulin lack. Unlike diabetic ketoacidosis, fasting ketosis is physiologic and a manifestation of appropriate metabolic regulation. It does not evolve into a severe condition similar to diabetic ketoacidosis, except potentially in a setting of severe volume depletion and metabolic stress.
Protein and Energy Metabolism
Muscle proteolysis is normally held under restraint by circulating insulin. As insulin levels fall in the postabsorptive state, this restraint is partially relaxed, and muscle proteolysis increases and exceeds protein synthesis. The free amino acids liberated by this imbalance (many of them first partially degraded to nonessential amino acids) enter the bloodstream and travel to the splanchnic organs for use in gluconeogenesis and protein synthesis. As the fast is extended, insulin levels fall yet lower, further increasing net muscle proteolysis. The loss of skeletal muscle from the body is considerable. During the first 7 to 10 days of a total fast, whole body nitrogen (N) loss is in the range of 10 to 12 g/day, excreted chiefly as urinary urea (42, 43). Because proteins are 16% N by weight and the lean tissues are 75% to 80% water, the loss of 10 to 12 g/day N from the body is equivalent to the loss of 300 to 400 g/day of lean tissue (42, 44). If this rate of body N loss were to continue, the body’s lean tissues would be lethally depleted within 3 weeks of continuous fasting. Instead, an adaptation takes effect after 7 to 10 days, which, by the end of 2 to 3 weeks, reduces the rate of N loss to less than one
half of its initial rate. This incompletely understood adaptation is all the more remarkable when it is appreciated that approximately one half of urinary N by this time is in the form of ammonium that has been synthesized to buffer the protons generated by ketoacid production (7, 45). In experiments in which ammonium excretion was reduced to normal by providing an exogenous buffer, the rate of body N loss in prolonged “adapted” fasting was close to the “obligatory” rate of N loss considered to indicate the maximum efficiency of endogenous protein turnover (46, 47, 48, 49).
half of its initial rate. This incompletely understood adaptation is all the more remarkable when it is appreciated that approximately one half of urinary N by this time is in the form of ammonium that has been synthesized to buffer the protons generated by ketoacid production (7, 45). In experiments in which ammonium excretion was reduced to normal by providing an exogenous buffer, the rate of body N loss in prolonged “adapted” fasting was close to the “obligatory” rate of N loss considered to indicate the maximum efficiency of endogenous protein turnover (46, 47, 48, 49).
Plasma branched-chain amino acid concentrations approximately double during the first 1 to 3 days of fasting, and their release from whole body proteins and subsequent oxidation increase by variable amounts (50, 51, 52). Urinary excretion of 3-methylhistidine, a marker of contractile protein breakdown, increases in the first few days of fasting (50, 53). However, by the 7- to 10-day mark, the initial increase in amino acid turnover is superseded, in some (50, 54) (although not all) studies (51), by a reduction in tracer-measured leucine or lysine (55) appearance into the circulation in a setting of continued significant urinary N excretion and whole body leucine oxidation. By the fourth week of fasting, urinary N excretion has greatly diminished, plasma leucine appearance further decreases (56, 57), and urinary 3-methylhistidine excretion is less than the prefasting rate (56).
The rapid rate of muscle protein loss in the first week of fasting is caused by a combination of reduced protein synthesis (owing to absent exogenous amino acids and the low-insulin state, because insulin normally stimulates protein synthesis) and the easing of insulin’s normal restraint on muscle proteolysis (15, 58). What process reverses this catabolic process after approximately 2 weeks of fasting? Most authorities regard the shift in muscle fuel preference from ketone bodies to fatty acids (and the resulting sparing of ketone bodies for use by the brain) as crucial. As ketone bodies increasingly displace glucose as the brain’s fuel, the body is not required to convert as much muscle protein into new glucose molecules, and the rate of net muscle proteolysis decreases.
But what is the specific metabolic signal that “tells” muscle cells to reduce their rate of net proteolysis at this point? Some studies suggest that hyperketonemia has a direct protein-sparing effect on skeletal muscle (59, 60), but an unequivocal demonstration of this effect is lacking (51). A role for free fatty acids in muscle protein sparing has been shown in short-term fasting humans (61). Perhaps increased fatty acid oxidation in muscle cells spares the branched-chain amino acids (which have structural similarity to fatty acids), and this sparing effect somehow mediates a reduction in net proteolysis (7, 62).
Weight Loss
During a prolonged total fast, both weight loss and N loss occur at a rate that is roughly proportional to the person’s existing body weight and lean body mass (63, 64). Nonobese men with free access to water may lose 4 kg during the first 5 days of fasting and a further 3 kg over the next 5 days (42, 65) whereas very obese men lose roughly 50% more than this. In one extreme case, a patient with an initial body weight of 245 kg lost 32 kg after 30 days of fasting (63).
Water, not fat, accounts for most of the weight loss that occurs early in fasting (66). Approximately 65% of the total water lost from the body during the first 3 days is extracellular (65). This rapid mobilization of extracellular water and sodium is partly caused by a lack of dietary sodium and partly by a drop in circulating insulin concentration, which reduces insulin-mediated renal tubular sodium reabsorption (67). There is also a rapid loss of intracellular water caused by the dissolution of lean tissues (19 to 25 g water/g N), liver glycogen (2 to 3 g water/g glycogen) (68), and to a lesser extent, partial depletion of muscle glycogen (3 to 4 g water/g glycogen) (44, 69). However, after 3 days, liver glycogen is either gone or stabilized, and by 2 weeks extracellular fluid balance has been restored (63). Weight loss consequently slows greatly, for it is now solely due to lean tissue and adipose tissue loss, and their rates of loss are themselves slowed by adaptive protein sparing and reduced energy expenditure. Weight loss by a 3-week fasting, moderately obese person is typically approximately 350 g/day. This rate of weight loss is both clinically observed also predictable by means of simple calculation. Thus, a negative N balance of 4 g/day is equivalent to the loss of 125 g/day of hydrated lean tissue. Because adipose tissue is approximately 85% pure fat (70, 71, 72), a negative energy balance of approximately 1700 kcal/day is equivalent to the loss of approximately 200 g/day of adipose tissue. The calculated total weight loss is 325 g/day. The rate of weight loss continues to slow further as the body’s lean tissue mass diminishes, in keeping with a first-order kinetic process.
Other Metabolic Effects
REE decreases within days of initiating a total fast; indeed, sleeping energy expenditure decreases within the first 48 hours (73). Some studies have reported a small increase in REE early in fasting (74, 75); this appears to result from catecholamine release that will occur if extracellular volume depletion is not prevented by generous sodium provision (76). After 2 weeks of fasting, REE has decreased by approximately 15% (77) and by 3 to 4 weeks by 25% to 35% lower than normal (65). The early reduction in REE is adaptive, being far too prompt to be accounted for by the loss of metabolically active tissues. Later reductions in REE are caused by the body’s decreasing metabolic mass.
Serum albumin concentrations remain normal both in short-term and prolonged fasting, but concentrations of the rapidly turning over liver secretory proteins, transthyretin (thyroid-binding prealbumin) and retinolbinding protein promptly fall, as occurs even after simple
carbohydrate restriction (78, 79). Ketonemia and extracellular volume depletion increase serum uric acid concentrations (10, 37). Serum total bilirubin typically increases by 50% after 24 hours of fasting and is often twice normal after 48 hours, but thereafter remains constant (80). Gastric emptying slows after 4 days of fasting (81). Mild chronic ketosis activates fetal hemoglobin production, and this may lead to a detectable increase in circulating fetal hemoglobin in some situations (82). Postural hypotension and nausea often occur in therapeutic fasts longer than 4 weeks, especially if table salt is not provided. Other metabolic effects and medical complications of prolonged fasting are described in clinical reviews (10, 63).
carbohydrate restriction (78, 79). Ketonemia and extracellular volume depletion increase serum uric acid concentrations (10, 37). Serum total bilirubin typically increases by 50% after 24 hours of fasting and is often twice normal after 48 hours, but thereafter remains constant (80). Gastric emptying slows after 4 days of fasting (81). Mild chronic ketosis activates fetal hemoglobin production, and this may lead to a detectable increase in circulating fetal hemoglobin in some situations (82). Postural hypotension and nausea often occur in therapeutic fasts longer than 4 weeks, especially if table salt is not provided. Other metabolic effects and medical complications of prolonged fasting are described in clinical reviews (10, 63).
Macronutrient Modifications of Fasting Metabolism
Carbohydrate provision blunts the early protein-catabolic phase of fasting, presumably by stimulating insulin secretion; fat has no similar protein-sparing effect (9, 54). As little as 100 to 150 g/day of glucose provision will prevent the ketonuria of fasting and reduce urea N excretion and extracellular volume loss by one half. For this reason, it is recommended clinical practice to infuse at least 1.5 L/day of intravenous solutions containing 5% dextrose in patients who must be acutely fasted (83). Carbohydrate’s protein-sparing effect is demonstrable during the first 7 to 10 days of a fast. When provided at later time points, even large amounts of carbohydrate only marginally reduce body N loss to less than the already low rate to which metabolic adaptation has brought it by this time (8, 84). By contrast, protein consumption has only a modest effect on body N loss during the first several days of fasting, but the provision of 50 to 80 g/day of high-quality protein will dramatically preserve body N stores in the long run. After a few days of generous protein provision, N balance improves and may even return to zero despite the ongoing negative energy balance (85, 86). When dietary protein is introduced for the first time in the late, adapted phase of a total fast, N balance promptly becomes positive despite the continuing strongly negative energy balance (56, 87). This phenomenon illustrates the entrainment of the adaptive mechanisms that increase the efficiency of endogenous protein turnover and increase the avidity with which dietary protein is retained.
Survival
The main determinant of survival in prolonged fasting is the size of the body’s initial fat store (88). Nonobese fasting adults die after approximately 60 days of continuous fasting (89), a time span consistent with complete loss of body fat but only approximately one third of the lean tissues (88, 90). Free fatty acids must be instantaneously available during prolonged fasting, for in this condition the brain depends on ketone bodies as well as glucose for energy. Fatty acid delivery provides the liver with substrate for ketone body synthesis as well as the fuel it needs to drive gluconeogenesis, which is an energyrequiring process (13). Fasting, therefore, is extremely hazardous for people whose fat stores are low even if their lean tissue stores are ample (91).
Obese individuals have tolerated fasts of astonishing length (92, 93). The longest well-documented fast on record was by a 27-year-old man with an initial weight of 207 kg who lost 60% of this weight after 382 days of uninterrupted fasting (94). Despite such spectacular reports, total fasts longer than approximately 4 weeks are potentially dangerous even for very obese people because, slow as it is, lean tissue loss does not cease. There are reports of extremely prolonged fasts in which, when lean tissue mass was measured, critical levels of depletion were observed even though the patients were asymptomatic (93). Acute thiamin deficiency is a devastating and entirely preventable complication both of prolonged fasting (95) and the refeeding phase following it (96).
PROTEIN DEFICIENCY
Minimum Protein Requirement
Pure protein deficiency comes about when protein intake is less than the body’s minimum requirement, but intake of the other essential nutrients, including energy, remains adequate. Pure protein deficiency is rare in clinical medicine. Its effects are of interest, however, because they bear on the definition and determination of minimum protein or essential amino acid requirements.
The normal response to a reduction in protein intake is an adaptive reduction in the rate of amino acid catabolism commensurate with the lowered intake and the restoration of zero N balance. The minimum requirement for protein (or an individual essential amino acid) has historically been understood as the lowest level of intake to which the body can adaptively reduce its catabolic rate to reestablish zero balance without incurring a physiologic cost (97, 98, 99). Although easy to conceptualize, this definition is extremely difficult to apply in practice. N balance promptly becomes negative whenever protein intake is reduced, then typically returns to zero after an adaptation period of a few days. Is the protein intake that produced this transient N loss “deficient”? The nineteenth century German physiologist Voit concluded that the protein requirement of normal men must be 120 g/day because he observed that their N balance became negative for the first few days after their protein intake was reduced to below this level. It is now appreciated that Voit’s research participants did not require a daily 120-g protein ration, they were merely habituated to it (100).
Proper understanding of human protein nutrition requires an appreciation of the transient and nutritionally trivial changes in body protein content that follow abrupt changes in the diet. Terms such as adaptation or normal adaptation have been proposed to describe normal
homeostatic adjustments to changes in protein intake that occur at or above the minimum requirement, and terms such as pathologic adaptation or accommodation to describe metabolic changes that restore N equilibrium, but only by means of important lean tissue sacrifice and at a physiologic cost. Adaptation is thus an aspect of normal homeostasis, whereas accommodation implies that homeostasis was reestablished at the expense of a physiologic compromise with adverse health implications (101).
homeostatic adjustments to changes in protein intake that occur at or above the minimum requirement, and terms such as pathologic adaptation or accommodation to describe metabolic changes that restore N equilibrium, but only by means of important lean tissue sacrifice and at a physiologic cost. Adaptation is thus an aspect of normal homeostasis, whereas accommodation implies that homeostasis was reestablished at the expense of a physiologic compromise with adverse health implications (101).
Protein Intakes Higher and Lower than the Requirement Level
Adaptive responses to changes in protein consumption that take place at more than the minimum requirement level are fundamentally different from the ones that occur when protein intake is decreased to less than this level (98). Essential amino acids are potentially highly toxic, so the body must promptly catabolize them at a rate equal to their rate of intake (102). Therefore, under equilibrium conditions, the body closely matches N loss to N intake, a metabolic agenda that calls for zero efficiency of amino acid retention. The transamination and oxidation rates of key amino acid catabolic enzymes increase linearly with increasing substrate concentration in the physiologic range (103). Surfeit amino acid consumption increases free amino acid pool sizes, automatically increasing their catabolic rate (104, 105). In confirmation of this understanding, whole body leucine oxidation has usually been found to be proportional to its plasma concentration (106). Beyond this “automatic” adaptation, however, increasingly high intakes of protein or specific essential amino acids induce adaptive increases in the mass or specific activity of pertinent catabolic enzymes, whereas reductions in intake have the opposite effect (107). The entrainment of these adaptive mechanisms, which may require as many as a few days, accounts for the transient increases and decreases in N balance that typically occur after abrupt increases and decreases in protein or essential amino acid intake which remain above the minimum requirement level.
The body’s metabolic agenda is entirely different when protein intake is reduced to less than the requirement level. In this situation, the body is called on to conserve dietary amino acids as efficiently as possible, and it does so by reducing proteolysis and increasing protein synthesis from dietary and endogenous amino acids. These adaptations reduce the size of the free amino acid pools and limit the increase that normally occurs in the fed state, thereby minimizing “overflow” catabolism. Amino acid catabolism is also minimized by adaptive decreases in the amounts or specific catalytic activities of key amino acid catabolic enzymes (101, 108).
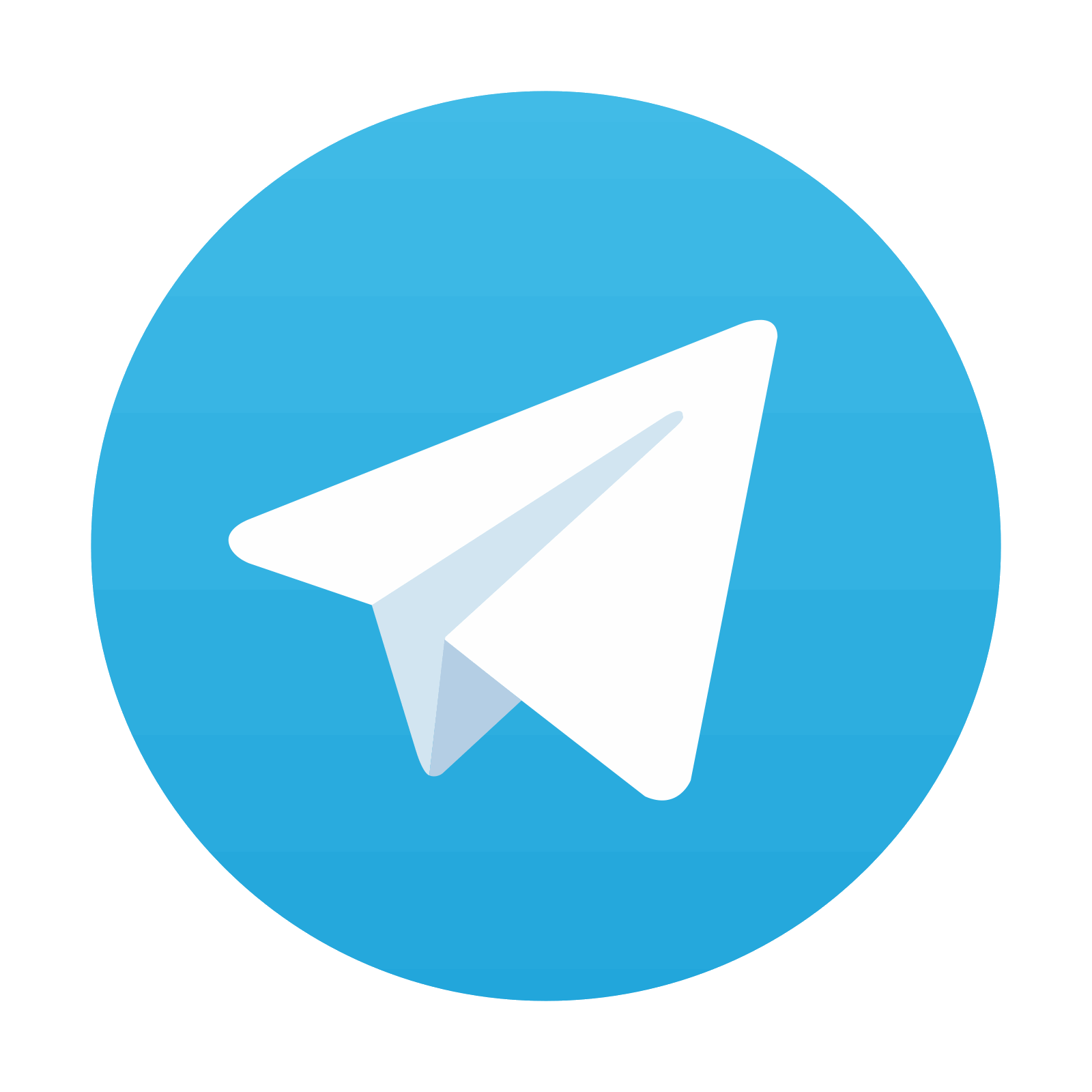
Stay updated, free articles. Join our Telegram channel
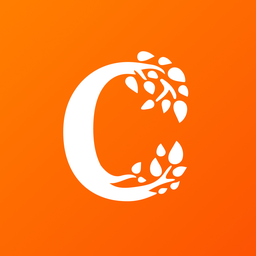
Full access? Get Clinical Tree
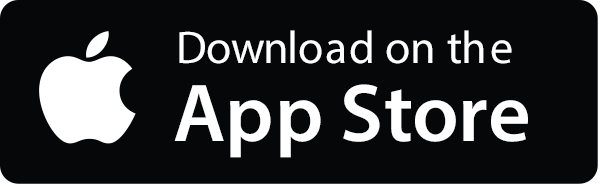
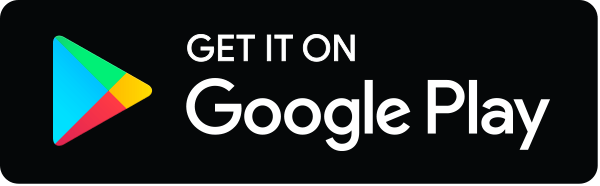