A. BACKGROUND
The purpose of this chapter is not to give a comprehensive description of membrane science (which would be impossible in one chapter) but rather to provide a brief overview perspective and to illustrate the connections to other areas of materials science.
Membranes play a significant but often unseen role in many important devices and processes. These range from the polymer electrolyte membranes discussed in Chapter 12 to the use of membranes in biomedical devices, described in Chapter 16, and include many other applications. In this chapter we review some general aspects of membrane science that apply to their uses for the separation of gases, liquids, or solutes, and for the controlled release of biologically active molecules. In addition, we briefly consider the use of membranes as acoustic transducers used in telephones or sonar equipment.
Many industrial processes would not be economically feasible without the use of membranes for chemical or biochemical separations. The same applies to the bio-medical sector, where the controlled release of pharmaceuticals is a key aspect of this technology. Many membranes are made from polymers, but some use ceramics, and a few make use of metals. There is also a close relationship between the chemistry of membranes and the science of surfaces (Chapter 15).
In general, a membrane is a thin film, plate, or wafer of material that separates two compartments of a device. A common feature of many membranes for separations and drug delivery is that they have special characteristics to optimize the transport of target molecules or ions across the material while, at the same time, retarding the transmission of other species. This selective transmission may be based on the molecular weight of the molecules being separated, including their size, shape, or compatibility or incompatibility with the membrane material. Such factors underlie the extraction of oxygen from air, the removal or organic pollutants from water, the separation of isotopes in gases, the removal of waste products from blood by hemodialysis, and the controlled delivery of drug molecules into the body. The use of membranes as sound transducers—to convert sound waves to electrical signals in microphones, or electrical signals to sound in speaker systems—is based on different principles that are considered at the end of this chapter.
Membranes can be divided into three classes—porous membranes, membranes that function by a chemical reaction between the membrane material and the target molecules, and membranes that are nonporous but transmit molecules by diffusion rather than chemical reactions.
1. Mechanism of Operation
Membranes that contains pores or tunnels that penetrate from one side of the material to the other are especially useful for gas separations and liquid filtrations. The membranes may be produced from inorganic oxides or from polymers. In ceramic porous membranes, the diffusion of gases is through rigid voids, tunnels, or galleries that penetrate the solid material. In such cases, diffusion may be controlled by the size of the pores, which restrict the gaseous flow of large molecules but allow smaller molecules to pass through. Alternatively, porous membranes may be used for liquid ultrafiltration. Pore sizes may range from nanometers to micrometers or larger, with the size in ceramic membranes controlled by synthesis techniques such as sol–gel methods, sintering of powders, removal of soluble template materials from within a composite solid, or electrochemical etching of an inorganic solid.
As an example, porous alumina membranes, with pore sizes in the micrometer–nanometer range, are produced by the acidic electrochemical oxidation of thin aluminum foils (Figure 13.1). Typically, pore diameters can range from 8 to 200 nm, with pore density in the range of 1011pores/cm2. For these structures to be used as membranes it is necessary to remove the unreacted aluminum foil that covers the closed ends of the tubular structure. Alumina porous membranes may also be used as templates for the deposition of metals in each pore. Dissolution of the alumina then leaves metal nanotubes or nanowires (see Chapter 17).
Porous polymer membranes are fabricated by (1) extracting one component of a polymer blend, (2) swelling the polymer in a solvent and then rapidly volatilizing the solvent, or (3) etching techniques. An example of process 3 involves exposure of a polymer membrane to α particles, which generate a path of damage through the material, a path that can be widened by etching with a reagent that penetrates preferentially along the damage track (Figure 13.2). Pore density then depends on the radiation exposure, and pore size is a function of the severity of etching. Such membranes provide a means for selecting the transmission of guest molecules on the basis of molecular size. Another approach is to produce a disk of stacked micro- or nanofibers, fill the space between them with a polymer, and then dissolve out the fibers. Alternatively, carbon nanotubes can be assembled in parallel arrays and used for nanoscale filtration. Tunnel clathrates and other nanoporous crystalline materials may also be adapted to produce porous membranes.
Figure 13.1. Porous aluminum oxide membranes can be produced by acidic electrochemical etching of the surface of aluminum foil.

Figure 13.2. Porous polymer membranes are produced commercially by exposure of a polymer film to α particles that penetrate the polymer, leaving behind a track of molecular damage. The damage track is more sensitive to etching by, for example, a strong base, which penetrates through the track and produces a cylindrical pore.

C. MEMBRANES THAT FUNCTION BY A CHEMICAL REACTION
Membranes of some metals, such as palladium, transmit hydrogen or deuterium at elevated temperatures (>300°C) but reject other gases. This behavior is now ascribed to the facile dissolution of hydrogen in the metal through the formation of a transient hydride. The alternative explanation, that hydrogen can diffuse through the free volume in the metal lattice, is now considered to be less likely. Evidence for the participation of metal hydrides is provided by the dramatic change in crystal structure that accompanies hydrogen absorption, a change that is consistent with the formation of metal hydrides. Separation processes based on this phenomenon are so effective that very large-scale industrial separations of hydrogen from other gases are accomplished by this technique. For example, the reaction products formed by heating carbon monoxide, methane, or other hydrocarbons with steam at 900°C are carbon monoxide, carbon dioxide, and hydrogen. The hydrogen can be isolated via palladium membranes. Interestingly, the alloying of about 20% silver with palladium yields a membrane that has an even higher permeability to hydrogen. The much heralded “hydrogen economy” could make extensive use of technology of this type of process.
D. NONPOROUS MEMBRANES THAT DO NOT REACT WITH PARTICIPATING MOLECULES
This is by far the largest class of membranes for gas or liquid separations and for the controlled release of small molecules. There are basically two different types of membranes that fall into this category—membranes fabricated from nonporous polymer films, and polymer matrices formed from a crosslinked polymer swollen by a solvent to form a gel. The distinction between these two types is sometimes unclear, but different mechanisms of operation are involved.
Polymer membranes are usually selective for the transmission of specific molecules because of three factors: (1) separation by differential solubility of the molecules (guests) at the surface of the membrane, known as “sorption”; (2) varied diffusion characteristics of different guest molecules or ions across the membrane; and (3) desorption of the molecules or ions at the other side of the membrane (Figure 13.3). In many gas or liquid separation systems, factors 1 and 2 have the most influence. For ion transport, all three factors are important.
Consider first the sorption factor at the leading face of a membrane. Gas or liquid molecules will interact with the surface of a membrane in different ways. Some will dissolve in the interface while others will not. In general, hydrophilic molecules will dissolve in a hydrophilic interface faster than will hydrophobic molecules, and vice versa. In practice, more is involved than a simple “like dissolves like” principle. Specific functional groups on one type of molecule may favor the attachment to and entry into a membrane surface. Some molecules may even react with the membrane material to give transient derivatives.
In addition, the ease of diffusion of specific molecules across a polymer membrane depends on several different factors. Two diffusion mechanisms are possible: one requiring diffusion through a solid polymer and the other involving diffusion through solvent molecules that are trapped within the membrane. In a solid polymer the guest molecules diffuse through the “free volume” between the polymer chains. Thus, the speed of diffusion depends on molecular size, the affinity of the guest for the host (strong interactions between the two may retard diffusion), and the freedom with which the polymer molecules can undergo conformational changes. Specifically, if the polymer is above its glass transition temperature, and the chains are able to undergo extensive molecular motions, the rate of guest diffusion will be faster than if the polymer is below the Tg. However, in practice, although the diffusion rate may be higher in a low-Tg polymer, the selectivity for transmission of different guests may be higher for a more rigid system that is below the Tg. Polymers above their Tg values may be less selective because the moving polymer chains allow both large and small molecules to diffuse through, whereas a rigid material may have relatively fixed and smaller “pore sizes.”
Figure 13.3. The transmission of molecules across a membrane can be understood in terms of three processes—sorption, diffusion, and desorption—with separations accomplished if different molecules in a mixture respond differently to any of these three processes.

In the case of membranes swollen by solvents, such as hydrogels or organogels Figure 13.4), the diffusion behavior may resemble the diffusion of molecules in solution, although the supporting polymer matrix can be an impediment to solute diffusion, especially if the degree of crosslinking is high or the membrane has a phase-separated structure. Thus, in gel membranes the relationship of the size of the diffusing species to the solvent-filled volume determines which molecules can penetrate the membrane and which cannot.
Figure 13.4. A hydrogel can function as a selection membrane if the free path through the crosslinked network allows some molecules to pass through, and others to be excluded.

Desorption of molecules that have traversed the membrane will be affected by mass-action effects. For example, if no guest molecules are present in the space beyond the membrane, desorption will be easy. If, on the other hand, a high concentration of guest exists on the far side of the membrane, desorption will be difficult. Hence, removal of guest molecules as soon as they have crossed the membrane is a key requirement for facilitating the process. To this end, a common practice for liquid separations is to apply pressure to the liquid on the entrant side of the membrane and apply a vacuum on the emergent side. The vacuum ensures rapid removal of molecules by evaporation as they emerge from the membrane, a process known as pervaporation.
E. SPECIFIC EXAMPLES OF MATERIALS USED IN SOLID POLYMERIC MEMBRANES
1. Poly(dimethylsiloxane) Membranes for Oxygen and Carbon Dioxide Transmission
Membranes of silicone rubber have a high permeability for oxygen and a moderately high permeability for carbon dioxide. For this reason they have been investigated for uses in underwater breathing equipment. A classical experiment illustrates this principle. Here, a mouse within an underwater chamber is separated from the water by a silicone rubber membrane. Dissolved oxygen passes from the water through the membrane to keep the mouse alive, while carbon dioxide diffuses in the opposite direction. The high gas permeability of silicone rubber is ascribed to two factors: (1) the unusually high solubility of oxygen in the polymer, for reasons not fully understood; and (2) the extensive molecular motion that exists at room temperature in silicone rubber, as indicated by its very low Tg (–130°C), generates a high free volume for guest transport. Other low-Tg polymers, such as polyphosphazenes with fluoroalkoxy side groups, also have good oxygen permeability characteristics.
2. Desalination Membranes
The removal of salts from seawater is a major engineering challenge. Among the methods employed for this purpose is the use of membranes for reverse osmosis. Seawater is pumped under pressure through a series of membranes that allow water to pass through but retard transmission of the salts. Traditional desalination membranes are nonporous polymers, but newer membranes are micro- or nanoporous materials through which the high-pressure water travels faster than dissolved salts
3. Dialysis Membranes
Dialysis is a liquid-phase separation process that allows small molecules to pass through a nonporous membrane while preventing the passage of larger molecules. Dialysis is used in polymer research to purify macromolecules from small-molecule impurities, and it is a major technique in biochemistry for the purification of proteins and other large biomolecules. Hemodialysis is a process by which blood is purified of low-molecular-weight waste products by the use of membranes that allow small molecules to pass through while, at the same time, preventing the loss of biological macromolecules such as proteins or larger biostructures such as blood corpuscles. Ideally the material from which the membrane is constructed should have a high selectivity and a good biocompatibility with blood. This subject is relevant to the biomedical materials discussion in Chapter 16.
The traditional dialysis membrane material is cellulose triacetate (produced from cellulose and acetic anhydride), which can be obtained with different “pore sizes” to allow the passage of molecules with specific dimensions. The term “pore size” is a misnomer for most dialysis membranes because no pores are present. Instead, the term “molecular weight cutoff” is more accurate since it defines the size of molecules that will and will not traverse the membrane. Cellulose acetate films are plasticized with glycerol and water to overcome the brittleness of the films. Thus, the diffusion of small molecules through the membrane is probably through the water- and glycerol-filled free volume between the polymer chains. In this sense, these membranes come close to the gel membranes described below. Molecular weight cutoffs can vary over a wide range, with values of 10,000–40,000 Da typical for protein dialysis. The different cutoff characteristics are achieved through a variety of proprietary techniques. In principle, the amount of solvent-filled free volume can be controlled by the level of acetylation of the cellulose and the amount of crosslinking.
4. Membranes for Controlled Drug Delivery
This topic is also revisited in Chapter 16. Controlled drug delivery is a process that releases a limited amount of a drug either to the skin for transdermal delivery or internally for release into the muscle system or bloodstream. The operating principle is that a polymer membrane is chosen that has a moderate permeability to the drug in question so that the release rate into the body is constant over a certain time period. Thus, the objective is not to separate molecules but to control their diffusion.
Typical devices consist of a reservoir of a drug in an aqueous medium, which is separated from the exterior by a membrane. Different drug molecules have different permeabilities through various membrane materials. Thus, membranes are chosen to provide a predetermined delivery rate, which often depends on the molecular size and hydrophilicity of the drug and the character of the membrane material. A long-existing polymer, poly(vinyl acetate), has been widely used for this purpose.
1. General Principles
A gel is a crosslinked polymer that is swollen by a solvent. Such materials can serve as reservoirs for the release of, for example, drug molecules, or they may be used as membranes for separations or for controlled drug delivery. The mechanism of action of a gel membrane usually depends on the diffusion of solutes through the liquid phase in the membrane. The polymer matrix may serve only to maintain the shape of the membrane. An exception was noted in Chapter 12, where the sulfonic acid groups attached to the polymer matrix in Nafion control the diffusion of protons through polymer electrolyte fuel cell membranes. The same may be true for the diffusion of lithium ions across a gel membrane in lithium polymer batteries. However, except for these special circumstances, the transmission of solute molecules through a gel membrane depends on the free path through the solvent component, and this, in turn, depends on the degree of cross-linking in the membrane.
2. The Special Case of Gel Membranes as On/Off Switching Systems
Numerous uses exist for membranes that can be switched from being permeable to being impermeable. An example is a device that automatically releases a drug molecule into the body in response to pH or temperature changes, or following the appearance of certain ions in the bloodstream. Many responsive membranes are solvent-swollen polymers that can expand or contract depending on an external stimulus. Some of the most interesting species in this category are hydrogels. In a water-swollen state a hydrogel membrane will allow the transmission of molecules through the water-filled volume. Some molecules may be excluded on the basis of the degree of crosslinking, with higher levels of crosslinking generating smaller free pathways for solute molecules to pass through.
However, if the gel collapses extruding the internal water, no solute molecules will pass through (Figure 13.5). There are four ways to bring about the collapse of a hydrogel: by changes in (1) temperature, (2) pH, and (3) cation charge, and by (4) the application of an electric potential. Hydrogels such as those formed from poly(ethylene oxide), poly(hydroxyethyl methacrylate) (HEMA), or the polyphosphazene MEEP (see Chapter 6, Table 6.1) have a lower critical solution temperature (LCST), which means that below a certain temperature they are fully expanded, but above that temperature they collapse and a membrane is no longer permeable. This behavior is a result of the balance of hydrophobic and hydrophilic components in the polymer and the change in hydrogen bonding to water as the temperature is raised.
Other hydrogels respond to pH changes. For example, a hydrogel formed from a water-soluble polymer with acidic side groups will be expanded at high pH but will collapse at low pH. The same gel will be expanded in solutions of monovalent cations, but will collapse through ionic crosslinking in the presence or di- or trivalent cations. Finally, if a gel derived from a polymer with acidic side groups contains cations that can be switched electrochemically from the monovalent to the di- or trivalent state, the passage of an electric current can bring about a reversible change in a membrane from permeable to impermeable. These changes also are illustrated in Figure 13.5. This same behavior can not only be used to control the passage of molecules through a membrane but also be employed to release molecules trapped in the hydrogel.
The evaluation of membranes is slightly different depending on whether a mixture to be separated is gaseous or liquid, and whether the membrane is designed for the controlled release of drug molecules.
Figure 13.5. Responsive hydrogel membranes derived from lightly crosslinked polyphosphazenes with both —OCH2CH2OCH2CH2OCH3 and OC6H4COOH side groups. The different curves are for polymers with different ratios of the two side groups. For example, polymer 4 contains 30% of the car-boxylate side groups while polymer 7 contains 94%. MEEP contains 100% of the alkyl ether side groups. The curve labeled “ester” is for a polymer where all the carboxylic acid groups have been esterified. (a) Contraction behavior of the hydorgels as the ionic strength of the aqueous medium is increased. (b) Contraction behavior as sodium ions in solution are replaced by calcium, and ferric ions. (c) Expansion of the membrane as the pH is increased. In the swollen state the membrane is permeable to small molecules. In the collapsed state, the pathways through the membrane are closed. These effects are all reversible. (From Allcock, H. R. and Ambrosio, A. M. A., Biomaterials, 17:2295–2302 (1996).

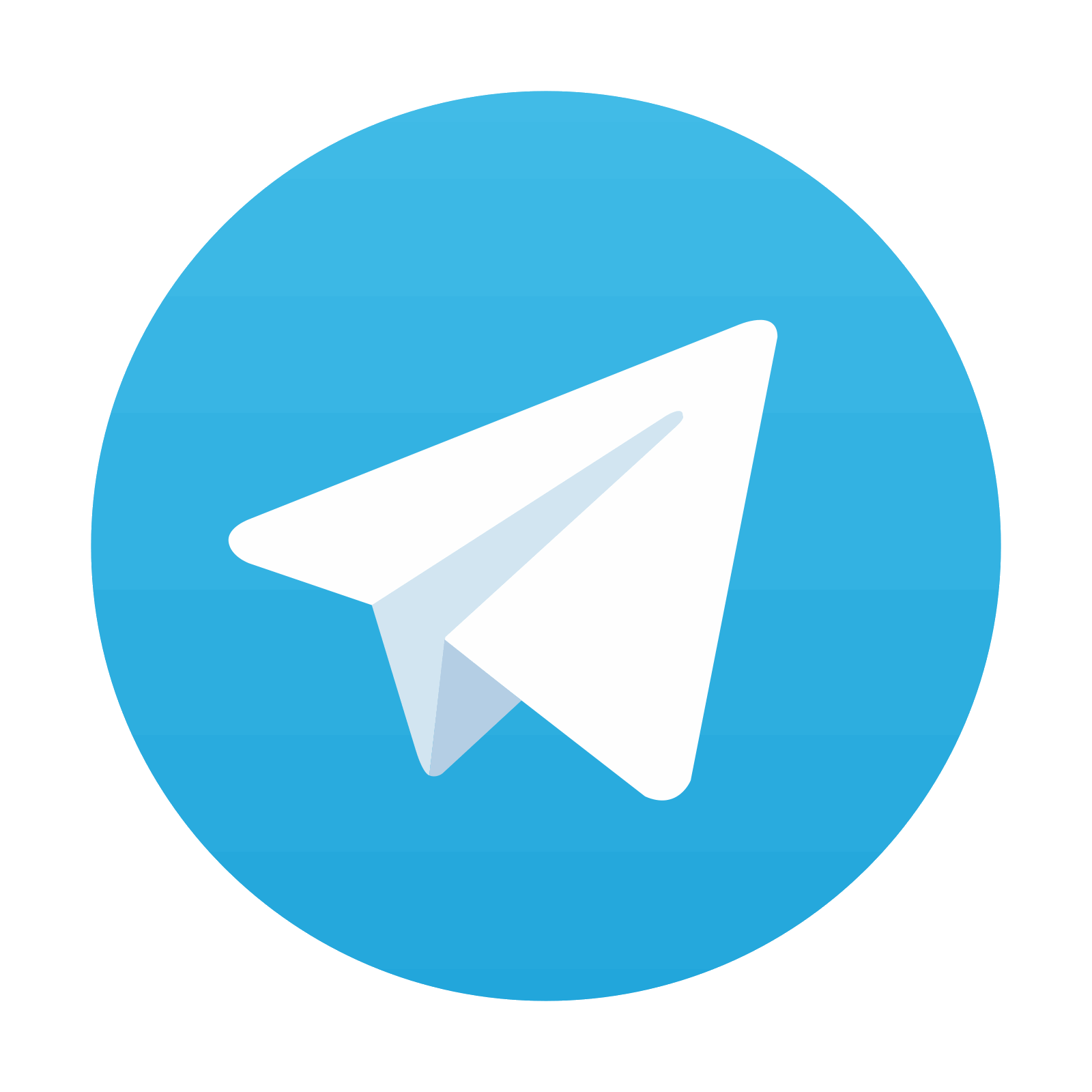
Stay updated, free articles. Join our Telegram channel
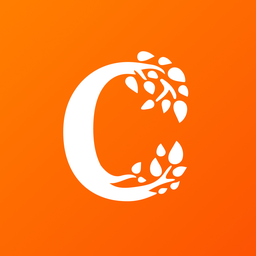
Full access? Get Clinical Tree
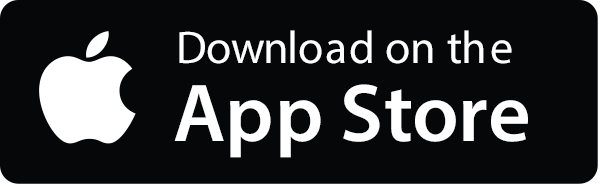
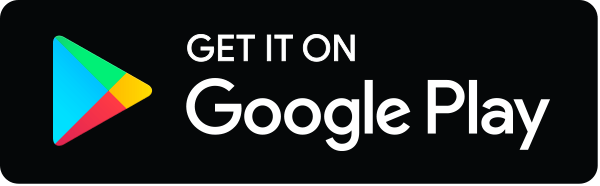