A. BACKGROUND AND MOTIVATION
The purpose of this chapter is to summarize the connections that exist between topics discussed earlier in the book and the rapidly expanding field of nanoscience. Thus, this is not a comprehensive survey of nanoscale materials science, but rather a general overview, and the reader is encouraged to use it as a starting point for further study.
Nanoscience and nanotechnology deal with objects that have dimensions in the range from 1 nanometer (10−9 meter) to about 100nm (1μm) (Table 17.1). Thus, nanostructures lie in the size regime between the Angstrom level (1 Å = 0.1 nm) and the microscale (from 1μm to roughly 1 mm). The Angstrom level is typically reserved for discussions of atom sizes, bond lengths, and small molecular structures. The microlevel is the range of integrated electronic circuits, living cells, and coarse texture in solids. Table 17.2 summarizes the classification of size features normally used by the materials science community. Nanoscale structures are a source of intense interest because new properties and unique behavior can be found when the size of objects falls below ~100nm. These new properties result because the ratio of surface area to volume is now so large that surface effects, such as those that give rise to catalysis, adsorption, and adhesion, become dominant characteristics. For example, a nanoparticle 5 nm in diameter has about half of its atoms at the surface. Moreover, electronic and photonic effects enter a new level because electrons are confined within such a small volume that quantum effects now dominate optical and electronic behavior.
Apart from the fundamental interest in these phenomena, nanoscience and nanotechnology are driven by the accelerating initiative to miniaturize devices. The development of smaller and smaller transistors in integrated circuits (see Chapter 10) provides an obvious example of the advantages of miniaturization in electronic technology. It is now reasoned that further miniaturization using nanoscale structures could bring about another technical revolution that will impact electronics and photonics, the treatment of diseases, and the development of new classes of ultra-high-performance materials that could propel technology in new directions.
TABLE 17.1. Size Regimes
1 micrometer (μm) | = one-millionth of a meter | 1 × 10−6 meter |
= one-thousandth of a millimeter | ||
1 nanometer (nm) | = one-billionth of a meter | 1 × 10−9 meter |
= one-thousandth of a micron | ||
1 angstrom (Å) | = one-tenth of a nanometer | 1 × 10−10 meter |
1 picometer (pm) | = one-hundredth of an Angstrom | 1 × 10−12 meter |
TABLE 17.2. Classification of Different Feature Sizes and Visualization

aSee Chapter 4.
The most obvious motivation for miniaturization below the macro- and microscales is the need for more rapid and more extensive information processing. As implied in Chapter 10, the performance of modern integrated electronic circuits is limited by the distances between transistors, the resistance of wiring between transistors, and the need to dissipate heat. Thus, smaller electronic circuits lead to faster processing, lower power consumption, and potential cost savings because the amount of silicon used per chip is correspondingly less. However, the miniaturization of optical circuits is an equally desirable objective for rapid information processing and transmission. Thus, nanoscale optical circuits might ovrcome many of the problems with electronic circuits. The miniaturization of medical devices, such as substrates for tissue engineering (Chapter 16), or controlled drug delivery through micellar units that can penetrate the smallest capillaries in the human vascular system, is an urgent need. Nanoscale machines that can penetrate into human cells to restore function or alter the replication process are being contemplated. Catalyst particles with nanoscale dimensions are crucial for many chemical and biochemical processes because of the high ratio of surface to volume. Two other areas of science and technology are fertile fields for the study of nanomaterials. The first includes the use of nanoscale powders of ceramics, polymers, and metals to form high-surface-area materials. The second involves the study of nanoscale domains in polymers, ceramics, and metals as a means to improve solid-state properties such as toughness and processing. In this respect, the “critical scale length”—the size below which new properties are generated—becomes a crucial factor in materials design.
Although widespread interest in nanoscience and nanotechnology is fairly recent, nanoscale structures have been known and studied for almost a century. Metallic colloids, for example, consist of nanoscale particles, as do some ceramic powders and precipitated polymers. However, the recognition that the size of particles, fibers, or surface features has an enormous influence on their properties is of fairly recent origin, and it underlies the current enthusiasm for all things “nano.” Specifically and realistically, the most important aspects of this field are connected with four issues. The first deals with the way in which nanoscale particles interact with their neighbors in solid arrays or with a second phase in composite materials. The second area of keen interest is the miniaturization of electronic and photonic circuits below the current microscale in order to pack an increasing number of features into an integrated array. This aspect brings forth the need to create nanowires to connect nanoscale transistors. Third, the development of methods to produce nanofibers is perhaps the most advanced area in terms of proximity to commercial success. Nanofibers are of great interest in biological tissue engineering, advanced textiles, and filtration technology. Fourth, the use of micelles, which have diameters in the nanorange, for controlled drug and vaccine delivery is a subject with a strong future. Applications of nanoscience in molecular machines or nano-robots, although prominent in the popular literature, are probably some distance over the horizon.
The continuing imperative toward miniaturization in nearly all areas of materials science presents numerous challenges for chemists, physicists, and engineers. These challenges range from the synthesis of nanoscale structures and their characterization to the manipulation and organization of them in two and three dimensions. The response of living cells to nanoscale objects is also a matter of growing interest.
B. SYNTHESIS AND FABRICATION OF NANOSTRUCTURES
Two different approaches exist for the preparation of nanostructures. These are known as the “top–down” and “bottom–up” methods. Top–down procedures start with micro- or macroscale materials, which are then broken down chemically or physically to nanoparticles. Methods that are used include grinding and abrasion (“ball milling”), chemical etching, electrospinning, and the vaporization of metals using plasmas or high-energy beams.
Bottom–up procedures involve the assembly of very small units (atoms, molecules, or small nanoparticles) to create larger constructs. It often happens that the units employed in the assembly process are formed by top–down procedures. Hence, the two approaches are intimately connected.
1. “Top–Down” Nanostructure Preparations
The grinding or ball milling of ceramics can reduce them to a fine powder with each individual particle having nanoscale dimensions. The main challenge with this method is to avoid contamination of the nanoparticles by the materials used in the abrasion process. A second disadvantage of this process is that the particle sizes are not uniform. This is not a problem if the particles are to be compressed and consolidated into a porous material, but it is a disadvantage if they are to be assembled into a uniform, regular, repeating three-dimensional array.
In general, polymers cannot be reduced to nanoparticles by grinding because of their molecular stucture (covalent bonds must be broken) and their impact resistance. An alternative is to precipitate a polymer from a solution into a nonsolvent. Under appropriate conditions it may be possible to form spherical particles with diameters below 100 nm. However, uniform polymer nanoparticles are also accessible via bottom–up techniques (see next section). Polymer fibers with diameters near or below 100 nm are produced by the process of electrospinning, as described in a later section.
In principle, nanostructures can be obtained through advanced photolithography techniques with the use of X rays or electron beams. Wavelengths below 100 nm would be needed to obtain the necessary resolution, although practical engineering restrictions provide serious hurdles.
2. “Bottom–Up” Synthesis Methods
Perhaps the simplest example of this approach is the compaction of nanoscale powders to form three-dimensional constructs. Uniform-sized particles, when assembled in this way, can yield regular repeating structures that resemble the atomic arrangements found in metals (see Chapter 8), but obviously on a larger scale. Another example is the assembly of molecules on a surface by moving them with an AFM probe.
Chemical synthesis can also yield nanoparticles. Indeed, some of the earliest nanoparticles recognized as such were metallic colloidal particles produced by reducing metal salts in solution by reagents such as formaldehyde. The size of the nanoparticles controls the color of the colloids so produced, with the larger particles giving a reddish color and the smallest providing a blue or green hue. Semiconductor nanoparticles have been produced by allowing a water-soluble cadmium salt to react with sodium sulfide in a polymer matrix which controls diffusion. However, this method often gives particles with a range of different diameters. Reactions carried out in sol–gel media are also capable of yielding nanostructures.
Chemical synthesis methods are also employed to produce polyhedral boranes and carboranes, fullerenes, and carbon nanotubes. For carbon-based structures, the syntheses depend on the volatilization of carbon atoms at high temperatures. These then recombine to form nanoscale cage molecules or, in the presence of metal catalysts, carbon nanotubes.
Self-assembly, another bottom–up technique, occurs when the shape of a molecule or nanostructure favors the formation of a regular repeating arrangement. An example is the way in which alkylthiol molecules coordinate to and assemble on a gold surface (see Chapter 15). The sulfur atom on each thiol molecule forms a coordinative attachment to the gold surface, while the alkyl chains assume a zigzag conformation and pack together with their neighbors to form a uniform pattern. This process can be employed to “image” a surface by means of photolithography of a resist attached to a gold surface. Alternatively, soft contact printing may be employed to lay down thiol molecules on discrete regions of the surface.
Self-assembly is also feasible when molecules enter and assemble within the galleries or pores of porous or layered intercalation solids or zeolites (Chapters 7 and 15) or in the tunnels of a clathrate adduct (Chapter 5). Biological structures such as proteins, nucleic acids, or the inorganic skeletal structures of microorganisms may also be used as templates for self-assembly.
Several of these methods for assembling nanoscale building blocks are discussed in more detail in the remainder of this chapter. The following sections cover a few specific examples where progress in nanoscience is readily apparent.
1. Nanofibers
The field of nanofibers has proved to be an area with broad advantages in both advanced technology and biomedicine. Nanofibers are produced mainly by the process of electrostatic spinning. The method is illustrated in Figure 6.7 (in Chapter 6).
As a reminder, in this process, a solution of a polymer in a hypodermic syringe is extruded through a needle by the action of a syringe pump. A direct current potential in the range of 15,000–30,000 V is connected between the metal needle and a metallic target. As each droplet of solution emerges slowly from the needle tip, it is attracted to the target by the potential difference, a process that induces thin fibers of solution to be spun out, accelerated, stretched, and deposited on the target. The solvent evaporates from the fiber during this process. The deposited nanofibers form a random fiber mat, which often resembles the appearance of tissue paper.
In addition, composite fibers can be spun by the use of two different polymers in solution or a suspension of nanoparticles in the polymer solution. Hollow nanofibers or fibers with a core that is different from the surface material are accessible by spinning two solutions through a needle that has concentric tubes. Moreover, the surface of the nanofibers can be smooth or rough depending on fabrication conditions.
In practice, very few polymers can be electrospun to dimensions that are less than 100 nm; most examples range within 100–300 nm. Nevertheless, even though these fibers are at the lower end of the microfiber ranges (Tables 17.1 and 17.2), they are usually described by researchers as nanofibers.
Three advantages of nanofibers over microfibers or films are as follows:
1. The overall surface roughness generated by the fiber mat brings about an increase in hydrophobicity, as indicated by a high water droplet contact angle (Chapter 15). For example, hydrophobic polymers have water contact angles near 100°. Nanofiber mats of the same polymer have contact angles of 155–160°, a dramatic increase in hydrophobicity brought about by the presence of air between the nanofibers.
2. The small diameter of nanofibers assists bonding to a second phase when the fibers are used for reinforcement in a polymer composite system.
3. Bioerodible polymer nanofiber mats are increasingly important in mammalian tissue regeneration. The small diameter of the fibers and the free space between them encourages the growth of cells in the form of a three-dimensional tissue. Tissue growth is encouraged by the “foothold” provided by the fibers, while the small diameter of each fiber accelerates bioerosion. An example is shown in Chapter 16 (Figure 16.6b).
Preceramic polymers (see Chapter 7) can also be electrospun, and the nanofibers obtained may be pyrolyzed to a nonoxide ceramic with the same nanofiber mat morphology as the precursor fibers. The use of ceramic nanofibers as reinforcement materials for ceramics and metals is a subject of considerable interest, because this provides a way to increase the strength of a host material in the same way that crystal growth in a metal or ceramic does. An example is the preparation of boron–carbon–silicon ceramic nanofibers from an organic-soluble polymeric precursor. Scanning electron micrographs of one such mat are shown in Chapter 7 as Figure 7.20. The different morphologies are obtained by changes in the concentrations of the solutions, the gap between needle tip and the target, and the rate of extrusion through the needle. The formation of nanofibers by electrospinning creates different morphologies depending on the concentration of polymer, the distance from the extrusion site to the target, and the voltage used. Fiber, beads distributed along nanofibers, and nanoscale beads alone are all accessible by changes to the spinning conditions.
2. Nanowires
Metal or conductive polymer nanowires may eventually be employed in electronic circuits. Silver nanowires have been produced by the reduction of solutions of silver salts. Although not fully understood why, it is known that the presence of poly(vinylpyrolidone) in the solution causes growth of silver mainly from the 100 face of the nanocrystals, and this generates nanofibers, some of which are hollow.
Nanorods are short nanowires that may be produced by metal vapor deposition into nanotubes that penetrate thin films of materials such as aluminum oxide (Chapter 13). Removal of the ceramic by etching in acid then releases the nanorods.
Nanowires can also be assembled from conductive or semiconductive nanoparticles by lining them up in contact with each other. Assembly methods that have been suggested include magnetic attraction, organization within nanochannels etched into a surface, or within tunnels that are α-track-etched through a membrane or that penetrate crystalline solids.
Silicon nanowires have been synthesized via chemical vapor deposition (CVD) techniques from silicon tetrachloride gas. One method utilizes a thin layer of gold nanoparticles deposited on a polymer surface. The nanoparticles act as “seeds” for the parallel growth of silicon nanowires from the surface in a CVD apparatus. The gold nanoparticles control the diameter of the fibers that grow from them. Patterning of the nanowire growth on a silicon surface using soft contact printing of the polymer, followed by deposition of gold nanoparticles, allows nanowires to be grown at strategic locations on a semiconductor chip.
3. Nanoscale Particles
a. Ceramic Nanospheres.
As described above, inorganic powders with radii below 100nm may be obtained by grinding (“sculpting”) from the bulk phase, by controlled precipitation or crystal growth, and by chemical reactions. Aerosol procedures have also been employed, as have sonochemical acoustic cavitation methods. Ceramic nanoparticles are raw materials used for consolidation to highly porous solids. Symmetrically packed nanospheres can be used as templates for the synthesis of porous materials. For example, a close-packed, three-dimensional assembly of ceramic nanospheres can be infused with a monomer, which polymerizes in the free space between the spheres. Decomposition of the spheres by acid or base then leaves a polymeric honeycomb (Figure 17.1). Honeycombs of this type have been proposed for a variety of uses such as filters, gas storage containers, and reservoirs for the controlled release of drugs.
Fluorescent silica nanoparticles are of interest for labeling biological molecules. In one report a fluorescent dye is first attached to a (sol–gel) silica precursor to form the core of a nanoparticle. This is then surrounded by a shell of sol–gel silica to give nanoparticles in the size range of 20–30 nm. Encapsulation of the dye in this way gives nanoparticles that fluoresce 20 times brighter than the unprotected dye because the surrounding liquid medium cannot interfere with the fluorescence process. Attachment of biological molecules to the outer shell then allows the location of the nanoparticles to be monitored within a living system by their fluorescence.
Figure 17.1. Formation of nanoscale honeycomb structures. (a) Nanospheres of ceramic, polymer, or metal are allowed to form a “crystal”-packing arrangement. (b) The space between nanospheres is filled with a polymerizable liquid (inorganic or organic), which, after polymerization, binds the structure together. (c) The nanospheres are then removed by strong acid or base (ceramic or metal spheres) or pyrolysis (polymer spheres) to leave a honeycomb of polymer or ceramic material.

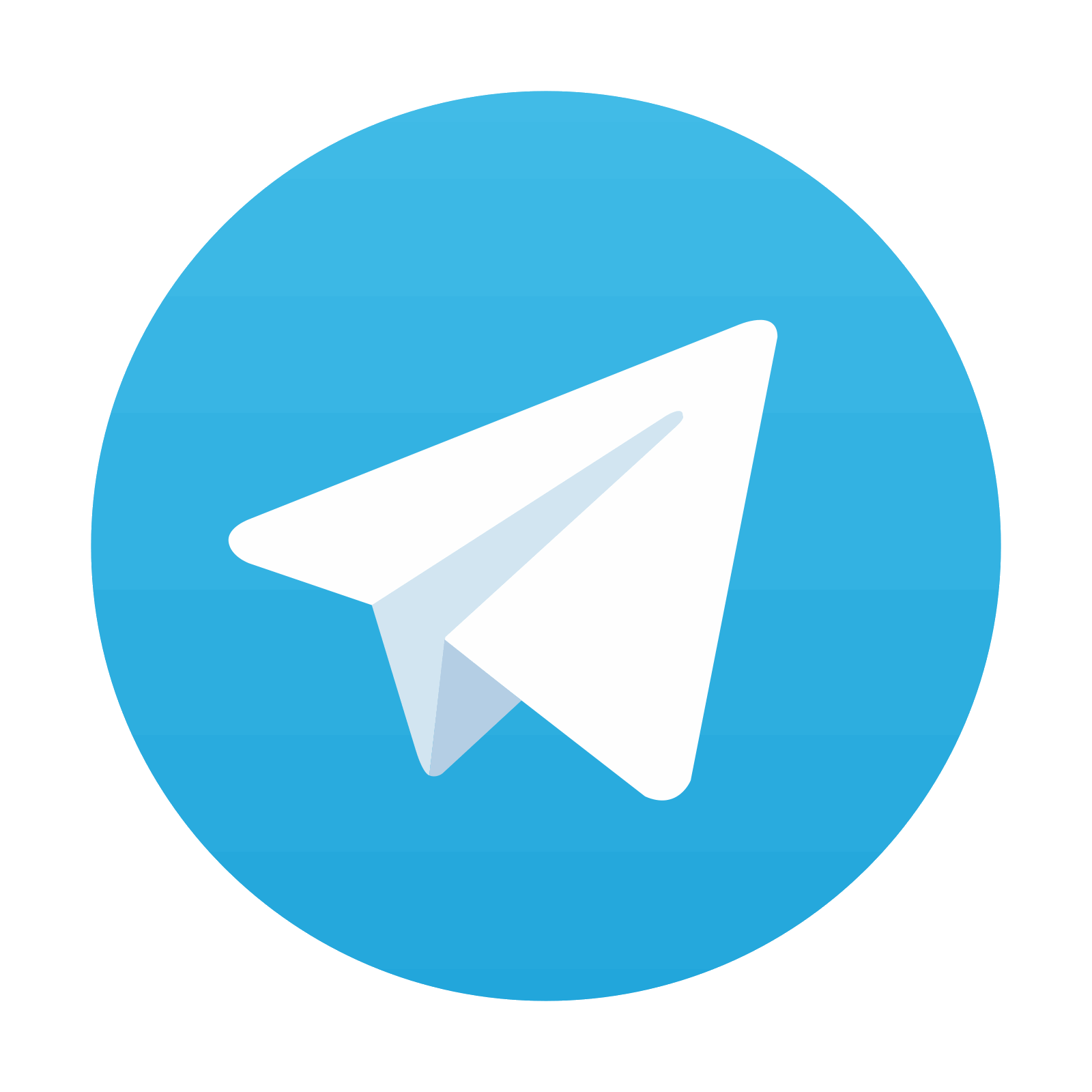
Stay updated, free articles. Join our Telegram channel
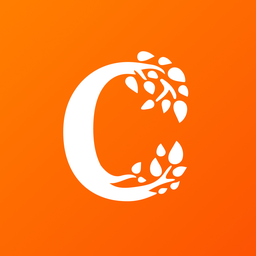
Full access? Get Clinical Tree
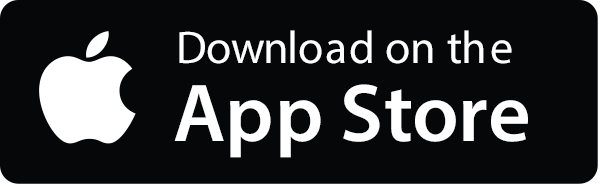
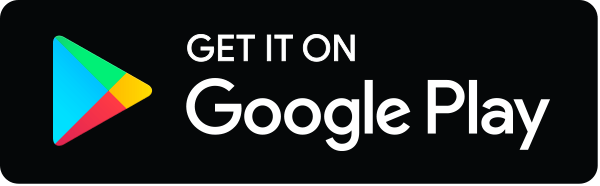