• Its action as an enzyme cofactor • Its ability to chelate anionic ligands, especially adenosine triphosphate (ATP) • Its ability to compete with calcium (Ca2+) for binding sites, which modulates intracellular and extracellular free Ca2+ concentrations • Its ability to cross-link negatively charged membrane constituents, which stabilizes them Intestinal Mg2+ absorption is inversely proportional to the amount ingested. Under normal dietary conditions in healthy individuals, approximately 30% to 50% of ingested Mg2+ is absorbed (Fine et al., 1991). Mg2+ is absorbed along the entire intestinal tract, but the sites of maximal Mg2+ absorption appear to be the distal jejunum and the ileum. The colon absorbs only small amounts of Mg2+, which may be important in the context of dietary restriction or compromised Mg2+ absorption in the small intestine. When dietary intake is restricted, fractional absorption of Mg2+ may increase up to 80%. Conversely, it may be reduced to 20% with a diet high in Mg2+. There is evidence that intestinal Mg2+ absorption is through paracellular and transcellular pathways (Quamme, 2008) (Figure 33-1). About 90% of normal Mg2+ absorption occurs passively through the paracellular pathway between the enterocytes, involving barrier proteins of the claudin family (claudin-16, claudin-19). The rate of Mg2+ absorption across the intestinal epithelium is dependent on the transepithelial electrical voltage (which is normally about +5 mV, lumen positive with respect to blood) and the transepithelial concentration gradient. The luminal Mg2+ concentration may be about 1.0 to 5.0 mM, depending on the dietary Mg2+ content and the presence of anionic chelators. Only the free Mg2+ moves through the paracellular pathway so that bound Mg2+ does not contribute to the transepithelial gradient. Plasma Mg2+ concentration is 0.5 to 0.7 mM, so there is normally a concentration gradient between the lumen and the blood. Various poorly understood hormonal and nonhormonal factors acting through a variety of intracellular signals might influence the passive transport. At a low intraluminal Mg2+ concentration, however, absorption occurs primarily via the active transcellular route. Transcellular Mg2+ absorption is an active system where the Mg2+ entry is mainly mediated by the TRPM6 (transient receptor potential melastatin) channel kinase (see Figure 33-1). TRPM6 protein is localized to the apical membrane of the intestine, thus forming the entry pathway into the enterocyte. Low Mg2+ intake induces an increased expression of TRPM6, facilitating Mg2+ absorption (Groenestege et al., 2006). The basolateral exit of Mg2+ is less well understood. Most probably it consists of a Na+/Mg2+ antiport system. In addition to these transporters, several other Mg2+-sensitive genes have been described that are expressed in intestine and are probably involved in Mg2+ transport (Quamme, 2008). Intestinal Mg2+ absorption can be affected by the presence of other nutrients and dietary constituents. High levels of dietary fiber from fruits, vegetables, and grains decrease fractional Mg2+ absorption (Siener and Hesse, 1995). However, diets high in vegetables are Mg2+-rich, and the high Mg2+ content of these diets offsets decreased fractional absorption associated with the higher fiber intake. Many foods high in fiber also contain phytate, which may decrease intestinal Mg2+ absorption because Mg2+ binds to the phosphate groups on phytic acid. The ability of phosphate to bind Mg2+ may explain decreases in intestinal Mg2+ absorption in individuals on high-phosphate diets (Franz, 1989). Although dietary calcium has been reported to both decrease and increase Mg2+ absorption, human studies have shown no effect (Fine et al., 1991). It is likely that interactions between Ca2+ and Mg2+ occur at high local concentrations achieved with Mg2+ or Ca2+ supplements but not with usual dietary supply. The kidney is the principal organ involved in Mg2+ homeostasis (Dimke et al., 2009). Under normal conditions, approximately 80% of the total plasma Mg2+ is filtered through the glomerulus, and most of this is reabsorbed (greater than 95%) as the filtrate passes through the nephrons (Figure 33-2). Therefore each day, only about 100 mg Mg2+ is excreted in the urine, whereas about 2,500 mg of Mg2+ is filtered by the glomeruli. The major site of Mg2+ reabsorption is the cortical segment of the thick ascending limb of the loop of Henle, which accounts for 65% to 75% of renal Mg2+ reabsorption. This Mg2+ transport is passive and involves claudin-16, with movement from the tubular lumen to the interstitium driven by the lumen-positive transepithelial voltage (Figure 33-3, A). The transepithelial voltage is determined by Na+, K+, and Cl– cotransport and active Na+ reabsorption. Therefore any changes in these transport mechanisms consequently influence Mg2+ (and Ca2+) reabsorption. Mg2+ reabsorption in the cortical thick ascending limb of the loop of Henle is regulated by a variety of hormones that act mainly by increasing the reabsorption rate. These hormonal responses are mediated by changes in both transepithelial voltage and paracellular permeability. It has been shown that 1,25-dihydroxyvitamin D influences Mg2+ transport in the cortical thick ascending limb by downregulating claudin-16 expression at the transcriptional level (Efrati et al., 2005). The amount of reabsorbed Mg2+ is mediated by the extracellular Ca2+/Mg2+-sensing receptor (CaSR), which is expressed in the basolateral membrane. Activation of CaSR by increased blood concentrations of Ca2+ or Mg2+ leads to inhibition of salt reabsorption and paracellular Ca2+ and Mg2+ transport in the thick ascending limb, thereby increasing divalent cation excretion. Loop diuretics such as furosemide, which act to inhibit the Cl– pump and subsequently block Na+ reabsorption, may lead to hypomagnesemia, as these drugs have a large effect on transepithelial voltage. Mg2+ reabsorption in the distal convoluted tubule is about 5% to 10% and is of crucial importance for regulating the final amount of excreted Mg2+, because there is no evidence of Mg2+ reabsorption beyond this point. Reabsorption is mediated by an active transcellular transport mechanism, as shown in Figure 33-3, B. Mg2+ enters the cell across the apical membrane through the TRPM6 channel. Uptake of Mg2+ is driven by the lumen-negative potential difference in this region of the tubule. Extrusion into the interstitium probably occurs by a Na+-dependent exchange mechanism. Mutations in TRPM6 were identified in patients with primary hypomagnesemia and with secondary hypocalcemia (Schlingmann et al., 2002; Walder et al., 2002). Mg2+ transport rates in the distal convoluted tubule change rapidly upon a decrease in Mg2+ availability, which allows efficient Mg2+ conservation. Conversely, during hypermagnesemia (or hypercalcemia), fractional excretion rates for Mg2+ are increased via activation of the CaSR, which results in inhibition of Mg2+ (or Ca2+) reabsorption. The epidermal growth factor (EGF) was identified as a magnesiotropic hormone. EGF stimulates the trafficking of TRPM6 channels to the luminal membrane, increasing the reabsorption of Mg2+ through TRPM6 (Thebault et al., 2009). Patients suffering from metastatic colorectal cancer are often treated with antibodies raised against the EGF receptor, such as cetuximab. Anti–EGF receptor monoclonal antibody therapy has been shown to result in hypomagnesemia in a significant number of patients (Tejpar et al., 2007). In addition, changes in acid–base balance significantly influence Mg2+ status, because production of TRPM6 is reduced in acidosis (Nijenhuis et al., 2006). In human volunteers a higher dietary acid load increased urinary Mg2+ losses (Rylander et al., 2006). The normal adult total body Mg2+ content is approximately 24 g, or 1,000 mmol, and 50% to 60% of this total resides in bone (Figure 33-4). Part of this Mg2+ is on the surface of bone and is in equilibrium with the extracellular Mg2+. At reduced plasma concentrations Mg2+ can be rapidly released from the bone surface, and at increased plasma concentrations Mg2+ is bound to the surface (Elin, 1994). Based on investigations with the stable isotopes 25Mg2+ and 26Mg2+ in a kinetic model of Mg2+ metabolism in healthy men, 24% of the human total Mg2+ exchanges rapidly; of this, 79% turns over in 115 hours, representing probably the bone surface pool. The remaining part, which may represent plasma and easily accessible extracellular space, turns over in less than 9 hours (Sabatier et al., 2003). Therefore magnesium in bone represents a reservoir that buffers extracellular magnesium concentration. In humans this magnesium-buffering capacity is reduced with increasing age, because nearly half of the magnesium content of bone is lost over a lifetime (Vormann and Anke, 2002). Extracellular Mg2+ accounts for only about 1% of total body Mg2+. The normal serum Mg2+ concentration is 0.75 to 1.0 mmol/L (Weisinger and Bellorin-Font, 1998). Most of the plasma Mg2+, approximately 60% to 65%, is ionized or free Mg2+. Of the remaining 35% to 40%, 5% to 10% is complexed to anions such as phosphate, citrate, and sulfate, and 30% is bound to proteins (chiefly albumin). Soft tissue contains about one half of the total body Mg2+, approximately 470 mmol. The Mg2+ content of soft tissues varies between 2.5 and 9 mmol/kg wet tissue weight. In general, the higher the metabolic activity of the cell, the higher the Mg2+ content. Within the cell, significant amounts of Mg2+ are in the nucleus, mitochondria, and endoplasmic (or sarcoplasmic) reticulum as well as in the cytosol (Birch, 1993). Most of the Mg2+ is bound to proteins and other negatively charged molecules such as nucleoside triphosphates and diphosphates (e.g., ATP and ADP) and nucleic acids (e.g., RNA and DNA). In the cytoplasm, about 80% of the Mg2+ is complexed with ATP (Frausto da Silva and Williams, 1991). Only 1% to 5% of the total intracellular pool is free ionized Mg2+ (Romani et al., 1993). The concentration of free Mg2+ in the cytosol of mammalian cells has been reported to range from 0.2 to 1.0 mmol/L, but values vary with cell type and means of measurement. The free Mg2+ in the cell cytosol is maintained at a relatively constant level, even when the Mg2+ concentration in the extracellular fluid is experimentally varied above or below the physiological range (Romani, 2007). The relative constancy of the free Mg2+ concentration in the intracellular milieu is attributed to the limited permeability of the plasma membrane to Mg2+ and to the operation of specific Mg2+ transport systems that regulate the rates at which Mg2+ enters or leaves cells. Cellular Mg2+ transport has been studied intensively, and it is mediated by a variety of Mg2+ transport proteins in different human tissues (Quamme, 2010). In most tissues, Mg2+ influx is dependent on TRPM7. This channel is ubiquitously expressed, whereas TRPM6 is only found along the intestine, kidney nephron, lung, and testis. However, total intracellular Mg2+ content is determined by a balance of Mg2+ influx and Mg2+ efflux. Whereas Mg2+ influx systems are quite well characterized, much less is known about the mechanisms underlying Mg2+ transport out of the cell. Mg2+ mainly leaves the cell through a Na+/Mg2+ exchange mechanism, which depends on Na+,K+-ATPase activity (Wolf et al., 2008). Various other Mg2+ transport systems have been discovered in recent years; however, it is not known how they contribute to intracellular Mg2+ homeostasis (Quamme, 2010). It is also not known if polymorphisms of Mg2+ transporters influence Mg2+ status and affect Mg2+-sensitive diseases. The main biological role of Mg2+ in mammalian cells is anion charge neutralization (Romani, 2007; Cowan, 2002). Mg2+ is particularly found in association with organic polyphosphates such as nucleotide triphosphates and nucleotide diphosphates (e.g., ATP4– and ADP3–). Mg2+ is also found with other highly anionic species, including multisubstituted phosphates of sugars such as inositol triphosphate, nucleic acids (RNA and DNA), and some carboxylates (e.g., isocitrate-Mg2+ as substrate for isocitrate lyase; carboxylate groups on proteins). Mg2+ is normally bound between the β- and γ-phosphates of nucleotide triphosphates, such as ATP, and between the α- and β-phosphates of nucleotide diphosphates, such as ADP (Figure 33-5). This arrangement neutralizes the negative charge density on the ATP or other nucleotide triphosphates or diphosphates, and it facilitates binding of the nucleotide phosphate to the enzymes that use them as substrates. In most reactions in which Mg2+ is involved, it is present as a complex with a nucleotide triphosphate or diphosphate, which serves as the substrate. The Mg2+ in these complexes does not interact directly with the enzyme in most cases, but it is linked by the substrate in an enzyme–substrate–metal type of substrate-bridged complex. Hence Mg2+ plays a dominant role in all enzymatic reactions that require nucleotide triphosphates or diphosphates. This reaction type is widespread in metabolism. Mg2+ also is required for binding to some enzymes or other proteins to stabilize them in the active conformation or to induce the formation of a binding site or active site. By these effects, Mg2+ is involved in numerous steps in central pathways of carbohydrate, lipid, and protein metabolism and in mitochondrial ATP synthesis. In addition, Mg2+ associates with nucleic acids, in which the phosphate groups in the nucleotide chains render the polymers negatively charged overall. Mg2+ stabilizes bending of RNA or DNA into particular curved or folded structures. This presumably occurs because of the cross-linking of the oxyanion centers of the phosphate residues by the divalent cation. Many hormones, neurotransmitters, and other cellular effectors regulate cellular activity via the adenylate cyclase system. As is the case for other ATP-utilizing enzymes, the actual substrate for adenylate cyclase is Mg2+-ATP (Figure 33-6). There is also evidence for a Mg2+ binding site on adenylate cyclase, through which Mg2+ directly increases enzyme activity. Another group of hormones and neurotransmitters exert their effects by raising the ionized calcium (Ca2+) concentration in the cytosol of their target cells through the activation of the phosphoinositol cycle (see Figure 33-6). One of the principal mechanisms by which this is thought to occur is by receptor-mediated activation of phospholipase C, which hydrolyzes a specific phospholipid in the plasma membrane, PIP2 (phosphatidylinositol 4,5-bisphosphate), to yield two biologically active products, diacylglycerol and inositol 1,4,5-triphosphate (IP3). Diacylglycerol activates protein kinase C, and IP3 triggers calcium release from the endoplasmic reticulum. The IP3 is rapidly inactivated by dephosphorylation. It appears that Mg2+ is essential for the normal functioning of this phosphoinositol cycle because the kinase that forms the PIP2 and also the enzymes that inactivate IP3 require Mg2+ at physiological concentrations (Volpe et al., 1990). In contrast, higher Mg2+ concentrations may decrease intracellular Ca2+ by two mechanisms: (1) noncompetitive inhibition of IP3 binding to its receptor, and (2) inhibition of the release of Ca2+ via IP3-gated channels (Volpe et al., 1990). Mg2+ has been called “nature’s physiological calcium channel blocker” (Iseri and French, 1984). During Mg2+ depletion, intracellular Ca2+ rises. This may be caused by both uptake from extracellular Ca2+ and release from intracellular Ca2+ stores. Mg2+ has been demonstrated to decrease the inward Ca2+ flux through slow Ca2+ channels (O’Rourke, 1993; White and Hartzell, 1989). In addition, Mg2+ will decrease the transport of Ca2+ out of the endoplasmic reticulum into the cell cytosol. Consequently, because Ca2+ plays an important role in skeletal and smooth muscle contraction, a state of Mg2+ depletion may result in muscle cramps, hypertension, and coronary and cerebral vasospasm (Altura and Altura, 1995).
Magnesium
Absorption, Bioavailability, and Excretion of Magnesium
Intestinal Absorption
Bioavailability
Renal Magnesium Handling
Body Magnesium Content
Physiological Roles of Magnesium
Enzyme and ATP Cofactor
Second Messenger Systems
Ca2+ Channels
Magnesium
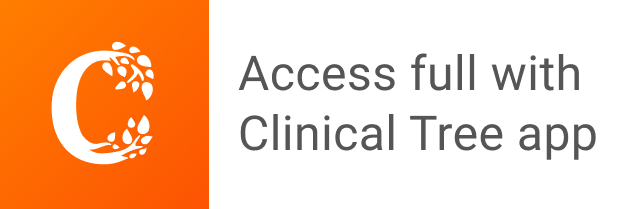