1. Recognition that replacing a hydrogen atom with a fluorine atom decreased flammability led to the introduction, in 1951, of the first halogenated hydrocarbon anesthetic, fluroxene.
2. Halothane was synthesized in 1951 and introduced for clinical use in 1956. However, the tendency for alkane derivatives such as halothane to enhance the arrhythmogenic effects of epinephrine led to the search for new inhaled anesthetics derived from ethers.
3. Methoxyflurane was introduced into clinical practice in 1960. Although methoxyflurane did not enhance the arrhythmogenic effects of epinephrine, its high solubility in blood and lipids resulted in a prolonged induction and slow recovery from anesthesia.
4. Enflurane, the next methyl ethyl ether derivative, was introduced for clinical use in 1973. This anesthetic, in contrast to halothane, does not enhance the arrhythmogenic effects of epinephrine or cause hepatotoxicity.
B. In search of a drug with fewer side effects, isoflurane, a structural isomer of enflurane, was introduced in 1981. This drug was resistant to metabolism, making organ toxicity unlikely after its administration.
II. Inhaled Anesthetics for the Present and Future
A. The search for even more pharmacologically “perfect” inhaled anesthetics did not end with the introduction and widespread use of isoflurane.
B. Desflurane, a totally fluorinated methyl ethyl ether, was introduced in 1992 and was followed in 1994 by the totally fluorinated methyl isopropyl ether, sevoflurane. The low solubility of these volatile anesthetics in blood facilitated rapid induction of anesthesia, precise control of end-tidal anesthetic concentrations during maintenance of anesthesia, and prompt recovery at the end of anesthesia independent of the duration of administration (important for ambulatory surgery).
C. Cost Considerations. The costs of new inhaled anesthetics can be decreased by using low, fresh gas flow rates. Less soluble anesthetics are more suitable for use with low gas flow rates because their poor solubility permits better control of the delivered concentration.
III. Current Clinically Useful Inhaled Anesthetics (Table 4-1) (Fig. 4-2)
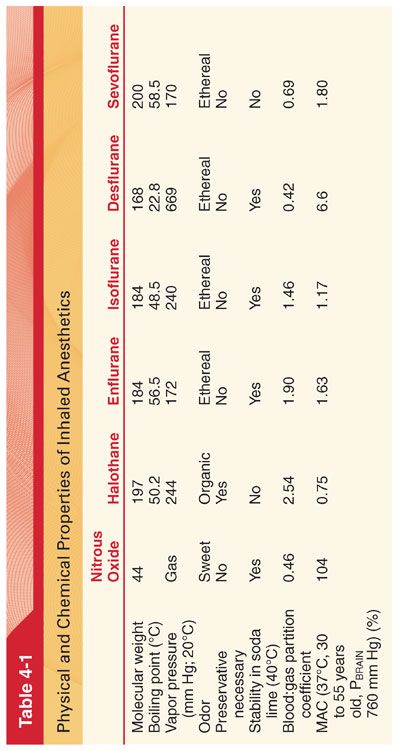
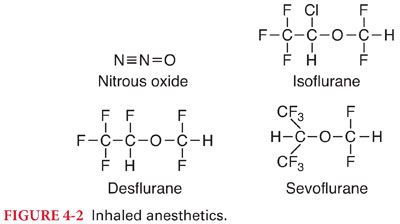
A. Nitrous oxide is a low-molecular-weight, odorless to sweet-smelling, nonflammable gas of low potency and poor blood solubility (blood:gas partition coefficient 0.46) that is most commonly administered in combination with opioids or volatile anesthetics to produce general anesthesia.
1. Although nitrous oxide is nonflammable, it will support combustion.
2. Its poor blood solubility permits rapid achievement of an alveolar and brain partial pressure of the drug (analgesic effects of nitrous oxide are prominent but short-lived) (Fig. 4-3).
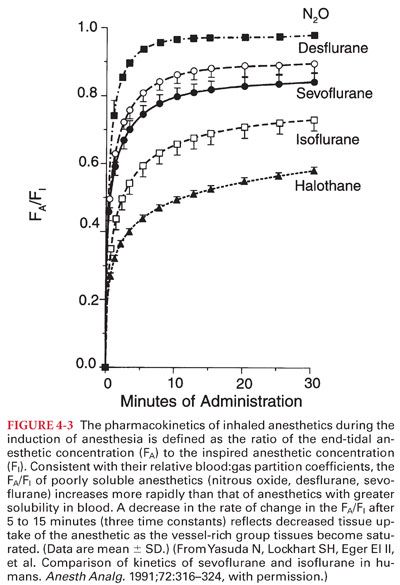
3. The benefits of nitrous oxide must be balanced against its possible adverse effects (high-volume absorption of nitrous oxide in gas containing spaces, potential increase in the risk of postoperative nausea and vomiting, its ability to inactivate vitamin B12).
B. Halothane, with its intermediate solubility in blood combined with a high potency, permits intermediate onset and recovery from anesthesia when administered alone or in combination with nitrous oxide or injected drugs such as opioids.
C. Enflurane, with its intermediate solubility in blood combined with a high potency, permits intermediate onset and recovery from anesthesia when administered alone or in combination with nitrous oxide or injected drugs such as opioids. Enflurane decreases the threshold for seizures (used for procedures in which a low threshold for seizure generation is desirable such as electroconvulsive therapy).
D. Isoflurane, with its intermediate solubility in blood combined with a high potency, permits intermediate onset and recovery from anesthesia using isoflurane alone or in combination with nitrous oxide or injected drugs such as opioids.
1. Although isoflurane is an isomer of enflurane, their manufacturing processes are not similar. The subsequent purification of isoflurane by distillation is complex and expensive.
2. Isoflurane is characterized by extreme physical stability.
E. Desflurane is a fluorinated methyl ethyl ether that differs from isoflurane only by substitution of a fluorine atom for the chlorine atom found on the α-ethyl component of isoflurane.
1. Fluorination rather than chlorination increases vapor pressure (decreases intermolecular attraction), enhances molecular stability, and decreases potency.
2. The vapor pressure of desflurane exceeds that of isoflurane by a factor of three such that desflurane would boil at normal operating room temperatures (requires a heated and pressurized vaporizer for delivery).
3. Unlike halothane and sevoflurane, desflurane is pungent, making it unlikely that inhalation induction of anesthesia will be feasible or pleasant for the patient.
4. Carbon monoxide results from degradation of desflurane by the strong base present in desiccated carbon dioxide absorbents.
5. Solubility characteristics (blood:gas partition coefficient 0.45) and potency (MAC 6.6%) permit rapid achievement of an alveolar partial pressure necessary for anesthesia followed by prompt awakening when desflurane is discontinued.
F. Sevoflurane is fluorinated methyl isopropyl ether.
1. The vapor pressure of sevoflurane resembles that of halothane and isoflurane, permitting delivery of this anesthetic via a conventional unheated vaporizer.
2. The solubility of sevoflurane (blood:gas partition coefficient 0.69) resembles that of desflurane, ensuring prompt induction of anesthesia and recovery after discontinuation of the anesthetic.
3. Sevoflurane is nonpungent, has minimal odor, produces bronchodilation similar in degree to isoflurane, and causes the least degree of airway irritation among the currently available volatile anesthetics (like halothane is acceptable for inhalation induction of anesthesia).
4. Sevoflurane may be 100-fold more vulnerable to metabolism than desflurane, with an estimated 3% to 5% of the dose undergoing biodegradation (fluoride).
5. Sevoflurane is the least likely volatile anesthetic to form carbon monoxide on exposure to carbon dioxide absorbents.
G. Xenon is an inert gas with many of the characteristics considered important for an ideal inhaled anesthetic (nonexplosive, nonpungent, odorless).
IV. Pharmacokinetics of inhaled anesthetics describes their (a) absorption (uptake) from alveoli into pulmonary capillary blood, (b) distribution in the body, (c) metabolism, and (d) elimination, principally via the lungs. A series of partial pressure gradients beginning at the anesthetic machine serve to propel the inhaled anesthetic across various barriers (alveoli, capillaries, cell membranes) to their sites of action in the central nervous system. The brain and all other tissues equilibrate with the partial pressures of inhaled anesthetics delivered to them by arterial blood (Pa).
A. Determinants of Alveolar Partial Pressure. The Pa and ultimately the PBRAIN of inhaled anesthetics are determined by input (delivery) into alveoli minus uptake (loss) of the drug from alveoli into arterial blood (Table 4-2).
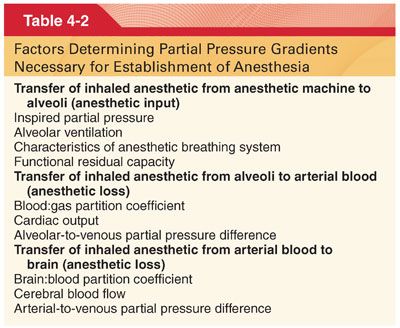
1. Inhaled Partial Pressure (PI). A high PI delivered from the anesthetic machine is required during initial administration of the anesthetic.
a. A high initial input offsets the impact of uptake, accelerating induction of anesthesia as reflected by the rate of rise in the Pa and thus the PBRAIN.
b. With time, as uptake into the blood decreases, the PI should be decreased to match the decreased anesthetic uptake and therefore maintain a constant and optimal PBRAIN.
2. Concentration Effect. The impact of PI on the rate of rise of the Pa of an inhaled anesthetic is known as the concentration effect (Fig. 4-4).
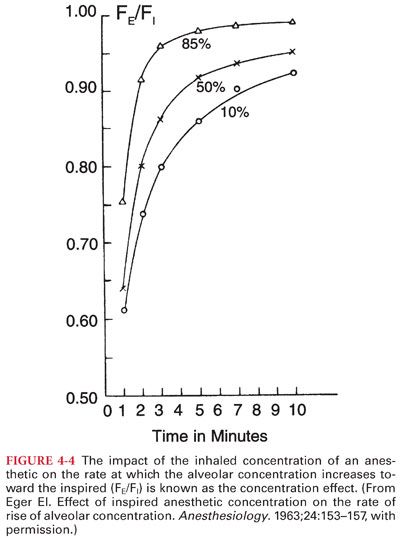
3. Second gas effect reflects the ability of high-volume uptake of one gas (first gas) to accelerate the rate of increase of the Pa of a concurrently administered “companion” gas (second gas) (Fig. 4-5).
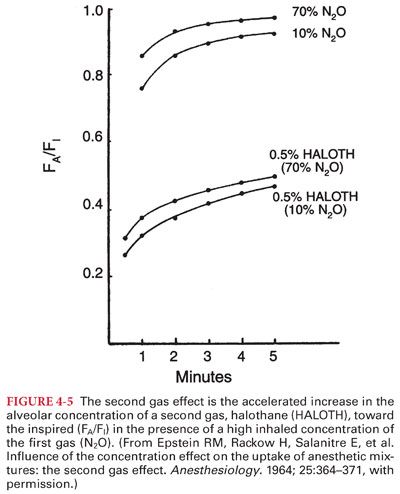
4. Spontaneous versus Mechanical Ventilation. Inhaled anesthetics influence their own uptake by virtue of dose-dependent depressant effects on alveolar ventilation. This, in effect, is a negative-feedback protective mechanism that prevents establishment of an excessive depth of anesthesia (delivery of anesthesia is decreased when ventilation is decreased) when a high PI is administered during spontaneous breathing (Fig. 4-6).
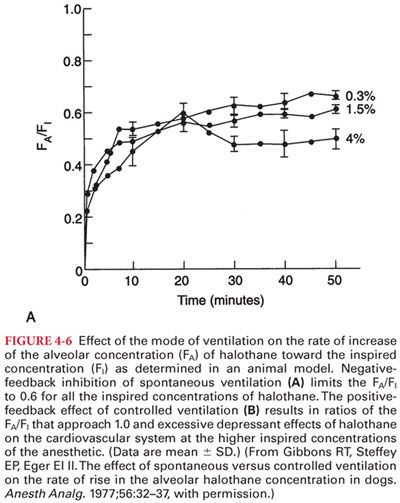
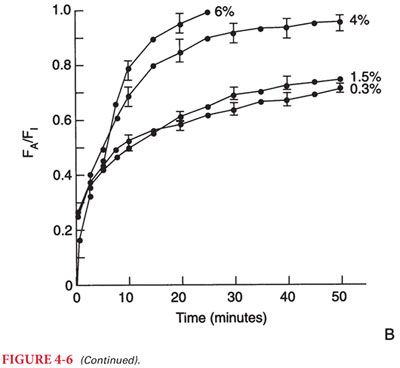
5. Impact of Solubility. The impact of changes in alveolar ventilation on the rate of increase in the Pa toward the PI depends on the solubility of the anesthetic in blood. For example, changes in alveolar ventilation influence the rate of increase of the Pa of a soluble anesthetic (halothane, isoflurane) more than a poorly soluble anesthetic (nitrous oxide, desflurane, sevoflurane). Indeed, the rate of increase in the Pa of nitrous oxide is rapid regardless of the alveolar ventilation.
6. Anesthetic Breathing System. Characteristics of the anesthetic breathing system that influence the rate of increase of the Pa are the (a) volume of the external breathing system, (b) solubility of the inhaled anesthetics in the rubber or plastic components of the breathing system, and (c) gas inflow from the anesthetic machine.
B. Solubility. The solubility of the inhaled anesthetics in blood and tissues is denoted by the partition coefficient (Table 4-3). A partition coefficient is a distribution ratio describing how the inhaled anesthetic distributes itself between two phases at equilibrium (partial pressures equal in both phases).
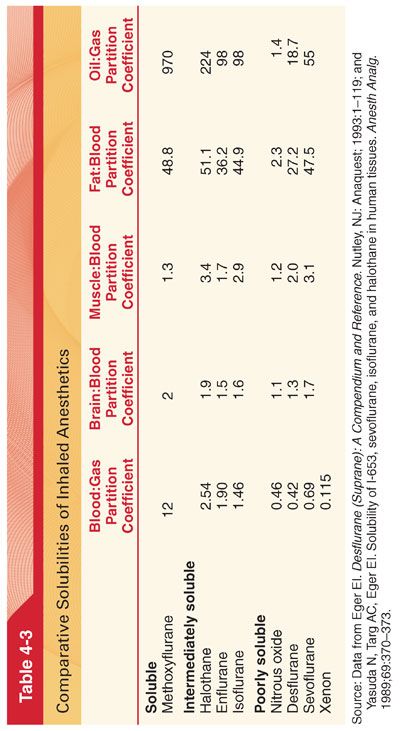
1. Blood:Gas Partition Coefficients. The rate of increase of the Pa toward the PI (maintained constant by mechanical ventilation of the lungs) is inversely related to the solubility of the anesthetic in blood (see Fig. 4-3).
2. Tissue:blood partition coefficients determine uptake of anesthetic into tissues and the time necessary for equilibration of tissues with the Pa.
a. The time for equilibration can be estimated by calculating a time constant (amount of inhaled anesthetic that can be dissolved in the tissue divided by tissue blood flow) for each tissue.
b. One time constant on an exponential curve represents 63% equilibration. Three time constants are equivalent to 95% equilibration. For volatile anesthetics, equilibration between the Pa and PBRAIN depends on the anesthetic’s blood solubility and requires 5 to 15 minutes (three time constants).
3. Nitrous Oxide Transfer to Closed Gas Spaces. The blood:gas partition coefficient of nitrous oxide (0.46) is about 34 times greater than that of nitrogen (0.014). This differential solubility means that nitrous oxide can leave the blood to enter an air-filled cavity 34 times more rapidly than nitrogen can leave the cavity to enter blood.
a. As a result of this preferential transfer of nitrous oxide, the volume or pressure of an air-filled cavity increases.
b. Passage of nitrous oxide into an air-filled cavity surrounded by a compliant wall (intestinal gas, pneumothorax, pulmonary blebs, air bubbles) causes the gas space to expand (Fig. 4-7). Conversely, passage of nitrous oxide into an air-filled cavity surrounded by a noncompliant wall (middle ear, cerebral ventricles, supratentorial space) causes an increase in intracavitary pressure.
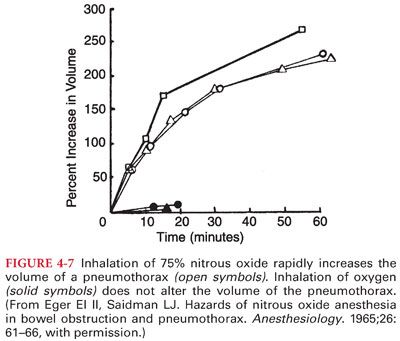
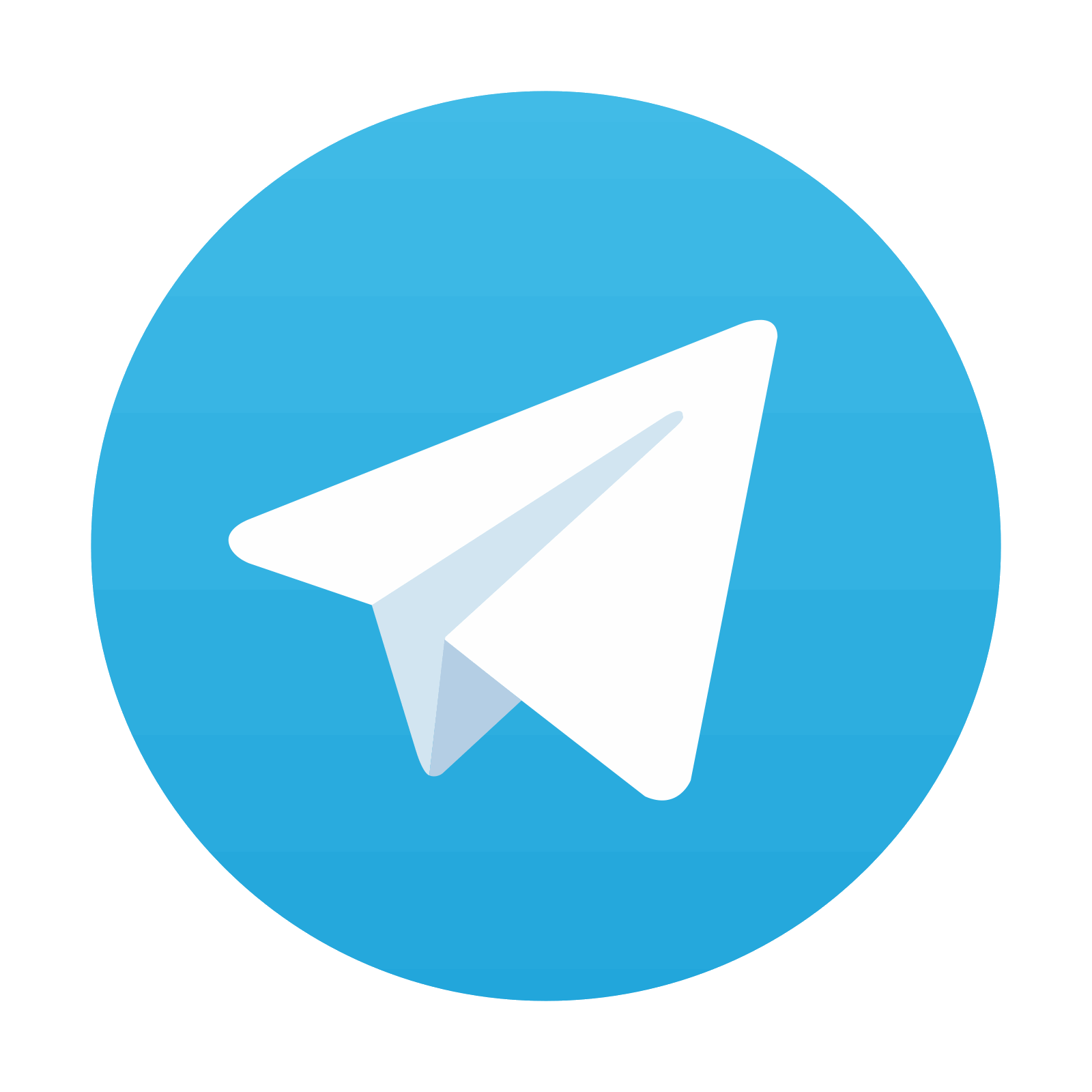
Stay updated, free articles. Join our Telegram channel
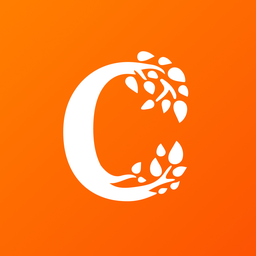
Full access? Get Clinical Tree
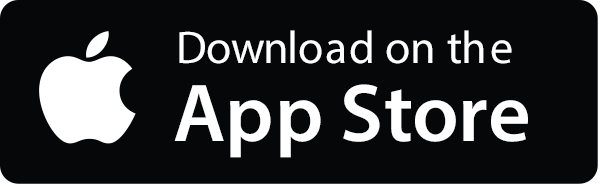
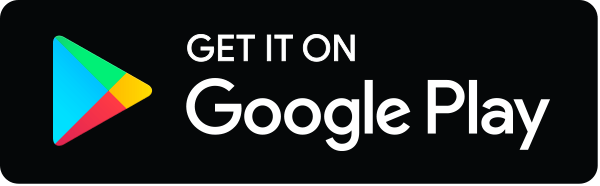