1.1 Introduction
The lung offers a unique and challenging route for drug delivery for the treatment of local respiratory and systemic diseases. Advances in drug formulation and inhalation device design are creating new opportunities for inhaled drug delivery as an alternative to oral and parenteral delivery methods. Nebulizers, pressurized metered-dose inhalers (pMDIs), and dry powder inhalers (DPIs) have each found a niche in the quest for optimal treatment and convenient use. While nebulizers have evolved relatively independently of the drug formulations they deliver, the current generation of pMDIs and DPIs have been developed or tailored for the specific pharmaceutical being delivered, resulting in improved performance. However, the process of delivering drugs to the lung is not simple and is related to many factors associated with the inhaled product and the patient. This chapter will briefly review the anatomy and physiology of the lungs and the various parameters that influence drug deposition.
1.2 Brief Review of the Respiratory System and its Physiology
The respiratory tract comprises the conducting and the respiratory regions. The conducting region essentially consists of the nasal cavity, nasopharynx, bronchi, and bronchioles. Airways distal to the bronchioles and the alveoli constitute the respiratory region, where rapid solute exchange takes place. According to Wiebel’s tracheobronchial classification [1], the conducting airways comprise the first 16 generations, and generations 17–23 include the respiratory bronchioles, the alveolar ducts, and the alveolar sacs.
The respiratory system is made up of a gas-exchanging organ (the lungs) and a pump that ventilates it. The pump consists of: the chest wall; the respiratory muscles, which increase and decrease the size of the thoracic cavity; the areas in the brain that control the muscles; and the tracts and nerves that connect the brain to the muscles. At rest, a normal human breathes 12–15 times a minute. About 500 mL of air per breath, or 6–8 L/min, is inspired and expired. This air mixes with the gas in the alveoli, and, by simple diffusion, O2 enters the blood in the pulmonary capillaries, while CO2 enters the alveoli. In this manner, 250 mL of O2 enters the body per minute and 200 mL of CO2 is excreted.
Anatomically, the respiratory system is divided into the upper and lower respiratory tract. The upper respiratory tract consists of the nose, pharynx, and larynx. The lower respiratory tract consists of the trachea, bronchial tree, and lungs. The human respiratory tract is made up of a series of bifurcating airways, starting at the oropharynx and finishing at the alveolar sacs. The airway anatomy consists of the oro, nasopharynx, larynx, trachea, two main bronchi, five lobar bronchi (three on the right, two on the left), and 15–20 dichotomous branchings of the bronchi and bronchioles down to the level of the terminal bronchioles and the alveoli. A schematic diagram of the human respiratory tract is given in Figure 1.1.
After passing through the nasal passages and pharynx, where it is warmed and takes up water vapor, the inspired air passes down the trachea and through the bronchioles, respiratory bronchioles, and alveolar ducts to the alveoli. The portion of the airways that participates in gas exchange with the pulmonary capillary blood consists of the respiratory bronchioles and the alveoli themselves. The alveoli act as the primary gas-exchange units of the lung, especially as the gas–blood barrier between the alveolar space and the pulmonary capillaries is extremely thin, allowing rapid gas exchange.
Between the trachea and the alveolar sacs, the airways divide 23 times. These multiple divisions greatly increase the total cross-sectional area of the airways, from 2.5 cm2 in the trachea to 11 800 cm2 in the alveoli [2].
Consequently, the velocity of airflow in the small airways declines to very low values. Oxygen can subsequently diffuse via the alveolar epithelium (a thin interstitial space) and the capillary endothelium. In simple terms, this provides a high surface area, low surface fluid coverage, thin diffusion layer, and sluggish cell surface clearance by macrophages. These properties provide an alternative delivery system to the more conventional gastrointestinal, nasal, buccal, or transdermal delivery routes [2]. Details of the anatomy and physiology of the respiratory tract are given in many texts; the reader is referred to Moren et al. [3] or a basic anatomy text [4].
1.3 Deposition and the Fate of Particles in the Respiratory Tract
The main factors here are the properties of the aerosol particles (particle size, aerodynamic diameter) and the mode of inspiration (breath volumes, flow rate) [5]. The most important parameter defining the site of deposition of aerosol drugs within the respiratory tract is the particle characteristics of the aerosol.
Most therapeutic aerosols are almost always heterodisperse, consisting of a wide range of particle sizes and described by the log-normal distribution with the log of the particle diameters plotted against particle number, surface area, or volume (mass) on a linear or probability scale and expressed as absolute values or cumulative percentage. Since delivered dose is very important when studying medical aerosols, particle number may be misleading, as smaller particles contain less drug than larger ones. Particle size is defined from this distribution by several parameters. The mass median aerodynamic diameter (MMAD) of an aerosol refers to the particle diameter that has 50% of the aerosol mass residing above and 50% below it. Strict control of MMAD of the particles ensures reproducibility of aerosol deposition and retention within desired regions of the respiratory tract. MMAD is read from the cumulative distribution curve at the 50% point. Geometric standard deviation (GSD) is a measure of the variability of the particle diameters within the aerosol and is calculated from the ratio of the particle diameter at the 84.1% point on the cumulative distribution curve to the MMAD. For a log-normal distribution, the GSD is the same for the number, surface area, or mass distributions. A GSD of 1 indicates a monodisperse aerosol, while a GSD of >1.2 indicates a heterodisperse aerosol.
The aerodynamic diameter relates the particle to the diameter of a sphere of unit density that has the same settling velocity as the particle of interest, regardless of its shape or density. Good distribution throughout the lung requires particles with an aerodynamic diameter between 1 and 5 μm, and thus most inhaled products are formulated with a high proportion of drug in this size range [6]. In order to target the alveolar region specifically, the aerosol droplet diameter should not be more than 3 μm. Particles with diameters that are greater than 6 μm are deposited in the oropharynx, whereas smaller particles (<1 μm) are exhaled during normal tidal breathing. Particles <2.5 μm are deposited mainly in the alveoli, where they can exert no pharmacodynamic effect and are rapidly absorbed, increasing the risk of systemic adverse events. This size range was confirmed for mild asthmatics, for whom the particle size of choice should be around 2.8 μm [7].
1.4 Deposition Mechanisms
As illustrated in Figure 1.2 (data from [8]), the deposition fractions of particles with different diameters in selected regions of the respiratory tract (laryngeal, upper and lower bronchial, alveolar) have been plotted as a function of particle size for particles of unit density (i.e. normalized aerodynamic diameter).
In this context, the term “deposition” refers to the mean probability of an inspired particle being deposited in the respiratory tract by collection on airway surfaces. “Total deposition” refers to particle collection in the whole respiratory tract, and “regional deposition” to particle collection in a particular region of the respiratory tract [8].
The delivery of medicaments to the respiratory tract is not a simple process. When particles do not follow airflow streamlines, coming in contact with surfaces, deposition occurs.
The entrainment and efficient delivery of particulates to the lung is regulated by three intrinsic physiological factors: inertial impaction, gravitational sedimentation, and diffusion (via Brownian motion) [9] (see Table 1.1).
Table 1.1 Deposition mechanisms in the lung and the Weibel model [10, 11]. Created using data with permission from J.S. Patton and P.R. Byron. Inhaling medicines: delivering drugs to the body through the lungs. Nature Reviews Drug Discovery. 6:67–74 (2007). 11. S.W. Clarke. Medical aerosol inhalers: past present and future. In S.W. Clarke and D. Pavia (eds.), Aerosola and the lung: Clinical and experimental aspects, Butterworths, London, 1984, pp. 1–18.
Although deposition occurs throughout the airways, inertial impaction usually occurs in the first 10 generations of the lung, where air velocity is high and airflow is turbulent [12]. Most particles >10 μm are deposited in the oropharyngeal region, with a large number impacting on the larynx, particularly when the drug is inhaled from devices requiring a high inspiratory flow rate (DPIs) or when it is dispensed from a device at a high forward velocity (MDIs) [13]. The large particles are subsequently swallowed and contribute minimally, if at all, to the therapeutic benefit.
Inertia is the inherent property of a moving mass that resists acceleration. It depends not only on the particle density and the particle diameter, but also on the airflow velocity.
Sedimentation is the gravitational settling of particles and mainly affects particles in the size range 1–8 μm. In this case, the distance that a particle will settle in a given time increases with the mass and is a gravity-dependent process. The longer a particle remains in the respiratory system, the larger the settling distance the particle covers and hence the greater the probability that the particle will get into contact with the airspace wall.
Brownian diffusion is the irregular motion of an aerosol particle in still air, caused by random variations in the incessant bombardment of gas molecules against the particles. It affects smaller particles (<1 μm), which deposit mainly in the alveolar region. In this area, air velocity is negligible, and thus the contribution to deposition by inertial impaction is nil. Particles in this region have a longer residence time and are deposited by both sedimentation and diffusion. Particles not deposited during inhalation are exhaled. Deposition due to sedimentation affects particles down to 0.5 μm in diameter, whereas below 0.5 μm, the main mechanism for deposition is diffusion.
In summary, minimal deposition occurs in the size range between 0.1 and 1 mm, because neither impaction, sedimentation, nor diffusion is effective in particle displacement. With decreasing particle diameter, diffusional particle displacement increases, so that particle deposition in the respiratory tract increases. With increasing particle diameter, the distance covered by sedimentation or impaction increases, so that the total particle deposition is also enhanced. The optimum size range of particulates for inhalation therapy has been shown to be 2.5–6 μm [2].
1.5 Parameters Influencing Particle Deposition
When designing and formulating a delivery system, the many factors that influence the deposition of drug particles need to be considered.
Increasing air velocity increases impaction deposition but decreases sedimentation and diffusion deposition. Breathing patterns – tidal volumes, respiratory time, and flow rates – all influence deposition. Total respiratory tract deposition increases with mean respiratory time and tidal volume (maximum inspiration volume). Total deposition has been shown to be dependent on the mean residence time (Tm) and the tidal volume (Vt) according to the following equation [14]:
(1.1)
where D is the diffusion coefficient of the particles in air. The respiratory period and mean residence time (Tm) of particles in the respiratory tract and the tidal volume (Vt) are the two most important breathing parameters affecting deposition of particles. Deposition increases to almost the same extent with an increase in Tm or Vt [14]. Age does not influence substantially the deposition patterns of particles, except perhaps for very small particles (1–2 nm) and for very young subjects (3 years old, in vitro cast data) [15]. There are inter-individual differences in the human population that affect the deposition and clearance, due to factors like age, existing respiratory conditions, state of the mucous layer, and exposure to other respiratory hazards (i.e. cigarette smoke) [16].
1.6 The Clearance of Deposited Particles
Like other parts of our organism, the lung has evolved to prevent the invasion of unwanted airborne particles into the body. Airway geometry, humidity, and clearance mechanisms contribute to this elimination process. The challenge in developing therapeutic aerosols is to produce one that eludes the lung’s various lines of defense.
1.7 Airways Geometry and Humidity
Progressive branching and narrowing of the airways encourages impaction of particles. The larger the particle size, the greater the velocity of incoming air, the greater the bend angle of bifurcations, and the smaller the airway radius, the greater the probability of deposition by impaction [17]. It must be remembered that in deposition studies, aerosol droplets and particles are highly dynamic systems [18]. The lung has a relative humidity of approximately 99.5%. Particle size does not remain constant once it reaches the respiratory tract. Volatile aerosols become smaller with evaporation, hygroscopic aerosols grow bigger with moisture from the respiratory tract, and particulates aerosols may agglomerate (Figure 1.3).
Figure 1.3 Hygroscopic growth and shrinkage of hypertonic and hypotonic droplets of the same initial size (3.7 μm) in the humid environment of the respiratory tract
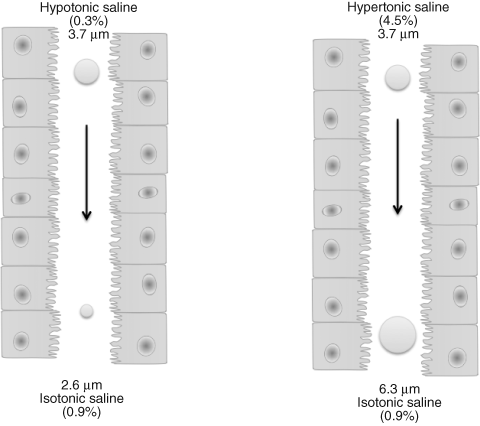
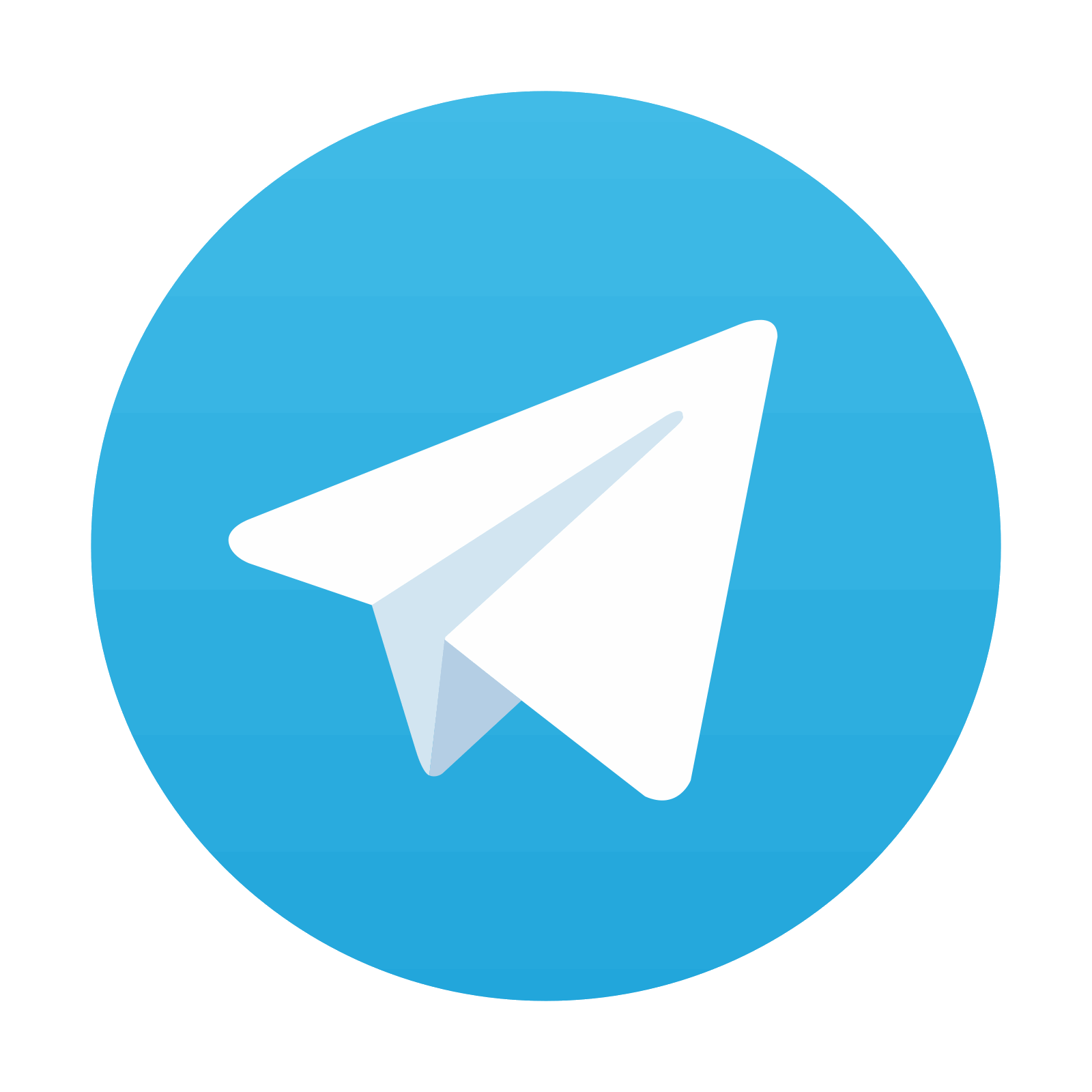
Stay updated, free articles. Join our Telegram channel
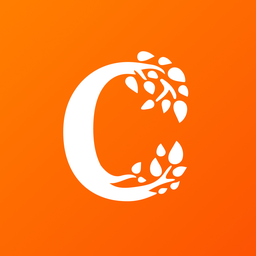
Full access? Get Clinical Tree
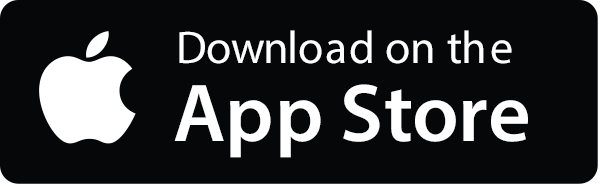
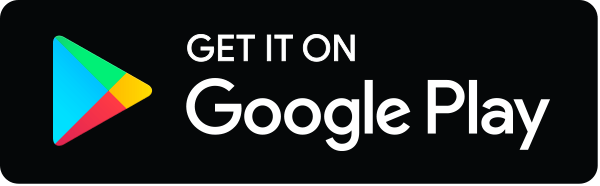