Improving the Water Solubility of Poorly Soluble Drugs |
CONTENTS
3.1.1 Biopharmaceutics Classification System
3.1.2 Strategies to Improve Water Solubility
3.2.2.2 Bottom-Up Technologies
3.6 Amorphous Solid Dispersions
3.6.1 Miscibility with Excipients
3.6.3 Improvement in Oral Absorption
3.8 Micellar Solubilization of Drugs
3.9 Oils, Emulsions, and Colloidal Carriers
3.9.1 Oils and Coarse Emulsions
3.9.2 Self-Microemulsifying Drug Delivery Systems
3.9.3 Other Colloidal Carriers
Ideally, drug development would involve the selection of active pharmaceutical ingredients (APIs) that possess ideal drug delivery characteristics, followed by their development using simple dosage forms. However, the reality is that increasingly formulators must work with APIs that have challenging physicochemical properties, including poor water solubility.
The increase in proportion of poorly soluble candidates is attributed to both improvements in synthesis technology, which has enabled the design of very complicated compounds, and also a change in focus in the discovery strategy of new APIs, from a so-called phenotypic approach to a target-based approach. The phenotypic approach involves trial-and-error methodology in which compounds are tested against cells, tissues, or the whole body. This approach takes into account various physicochemical and biological factors that may affect the efficacy of candidates, including solubility, protein binding, and metabolism. In the target-based approach, candidate compounds are screened against specific targets, based on hypotheses concerning action mechanisms. Lead compounds are typically dissolved in dimethyl sulfoxide for high-throughput screening (HTS), which means that even very poorly soluble drugs can be tested. Although the HTS approach provides a clear lead with respect to molecular design, compounds with poor aqueous solubility can progress to development after screening.
Poor water solubility has important ramifications for the drug discovery process, as poorly soluble lead compounds cannot be adequately formulated for subsequent preclinical studies in animals. Thus, it may not be possible to follow up potentially promising leads, which instead have to be dropped from the discovery process, never realizing their true potential. Although it may be possible to overcome the solubility problem by chemical modification of the drug, in many cases this is not feasible.
Poor water solubility also has important ramifications for drug bioavailability. In order to cross an epithelial interface, the drug must usually be dissolved in the biological fluids at that interface. For example, for the oral route, the first step in the oral absorption process is dissolution of the drug in the gastrointestinal (GI) lumen contents. A drug that is poorly soluble in the aqueous GI fluids will demonstrate poor and erratic dissolution, with concomitant low absorption and thus poor bioavailability—even if the drug possesses good intestinal permeability characteristics. Furthermore, the rate of intestinal absorption is driven by the concentration gradient between the intestinal lumen and the blood. A low concentration gradient is a poor driver for absorption, with a concomitant retarded flux across the intestinal epithelium.
As described in detail in Chapter 7, a significant hurdle associated with the oral route is the extreme variability in GI conditions, which can cause large intra- and interindividual variability in pharmacokinetic profiles. Poor water solubility exacerbates this variability, as there is a lack of dose proportionality for these compounds, as well as significant variability depending on the presence of food and fluids in the GI tract. The activity of bile salts on drug solubilization is a further important variable. These formulation and bioavailability concerns are equally relevant for poorly soluble drugs delivered via alternative epithelial routes, such as the pulmonary, topical, nasal, vaginal, and ocular routes.
3.1.1 BIOPHARMACEUTICS CLASSIFICATION SYSTEM
The Biopharmaceutics Classification System (BCS) classifies drugs into four categories, based on their aqueous solubility and ability to permeate the GI membrane (Figure 3.1). (However, it should be noted that the BCS is relevant to permeation across all biological membranes, not just the GI tract.) Based on pioneering work by Gordon Amidon at the University of Michigan (Amidon et al. 1995), the system has been adopted by the U.S. Food and Drug Administration (FDA) to allow pharmaceutical companies a waiver of clinical bioequivalence studies (a biowaiver), when seeking regulation of postapproval changes and generics. Increasingly, the BCS is being used as a tool in product development, to flag up potential solubility and permeability difficulties that may be associated with lead compounds.
A drug is considered highly soluble if its highest dose strength is soluble in less than 250 mL of water, as tested over a pH range of 1–7.5. A drug is considered high permeable if the oral absorption compares favorably (i.e., higher than 90%) to an intravenous injection of the drug. Absorption in vivo can be carried out by monitoring the appearance of the drug in the plasma after oral administration. Intestinal permeability may also be assessed by other methods, including in vivo intestinal perfusions studies in humans, in vivo or in situ intestinal perfusion studies in animals, in vitro permeation experiments with excised human or animal intestinal tissue, and in vitro permeation experiments across epithelial cell monolayers, such as the Caco-2 cell line.
FIGURE 3.1 The Biopharmaceutics Classification System. (Courtesy of Particle Sciences, Inc.)
Using the BCS, four distinct classes of drug can be defined as the following:
• Class I drugs possess characteristics that ensure good bioavailability: they dissolve rapidly in the GI fluids and then rapidly permeate the epithelial barrier.
• Drugs that fall into Class II possess good permeability characteristics, but they have poor solubility, which limits their bioavailability. Approximately 35%–40% of the top 200 drugs listed in the United States and other countries as immediate-release oral formulations are practically insoluble (see also Chapter 20, Figure 20.6). The bioavailability of a Class II drug can be markedly improved by improving its solubility: various methods to improve drug solubility are the focus of this chapter. The fact that about 40% of the top marketed drugs are practically insoluble, yet are nevertheless used commercially, is a testimony to the success of current solubilization methods.
• Class III drugs, although highly soluble, possess poor permeability. Permeability across epithelial barriers and strategies to improve epithelial permeability are described in Chapter 4.
• Class IV drugs have both poor solubility and permeability. In the case of Class IV drugs, improving the solubility may help somewhat toward improving bioavailability, although poor permeability will still be an issue.
3.1.2 STRATEGIES TO IMPROVE WATER SOLUBILITY
Poor solubility and permeability problems may be addressed at the chemical level, via lead optimization: this approach is described in Chapter 20 (Section 20.8). This chapter describes approaches used to increase the solubility of a poorly soluble API. A wide range of approaches can be used, as summarized in Table 3.1.
Which approach to use is partly determined by the nature of the drug. Poorly soluble drugs, i.e., Class II and Class IV of the BCS classification situation, can be further subclassified into two types of molecules (Bergström et al. 2007):
1. “Grease ball”: highly lipophilic compounds, with a high log P (>4) and a low melting point (<200°C). These compounds cannot form bonds with water molecules; thus, their solubility is limited by the solvation process.
2. “Brick dust”: compounds usually with low energy, highly stable crystal forms, with a high melting point (>200°C), and with poor water and lipid solubility (log P < 2). The water solubility of such compounds is restricted due to strong intermolecular bonds within the crystal structure.
TABLE 3.1
Mechanisms to Improve the Solubility of Poorly Soluble Active Pharmaceutical Ingredients
Approach | Example |
Physical modification | Micronization |
Nanosizing | |
Chemical modification | Prodrug formation |
Crystal engineering | Salt formation |
Polymorphs | |
Cocrystals | |
Formulation approaches | Solvent composition |
• Cosolvents • pH adjustment • Formulation excipients: surfactants, oils, etc. | |
ASDs | |
Cyclodextrin inclusion complexes | |
Colloidal systems | |
• Micelles • SMEDDS • Liposomes • Nano- and microparticulates | |
ASDs, amorphous solid dispersions; SMEDDS, self-microemulsifying drug delivery systems. |
The solubility of “grease ball” molecules can be increased if appropriate formulation strategies are used to overcome, or at least improve, the solvation process. A traditional approach for parenteral formulations is to administer the drug with a cosolvent: as a mixture of water with a water-miscible solvent such as propylene glycol, ethanol, and poly(ethylene glycol) (PEG) 300. However, even with the use of a cosolvent, it may only be possible to achieve low drug loading. Additionally, harsh vehicles such as organic solvents carry the risk of toxicity, particularly cardiotoxicity, in vivo. Precipitation of the drug on dilution with the body fluids may also occur, causing pain and inflammation at the injection site, as well as the possibility of emboli. For intramuscular delivery, precipitation of the formulation may result in the formulation acting more like a depot injection, resulting in the delayed absorption of the drug. Other formulation strategies for “grease ball” molecules include the use of cyclodextrin (CD) inclusion complexes, and the use of micelle and emulsion-based delivery systems. In contrast, “brick dust” molecules are not only poorly soluble in water, but also in oils, rendering them unsuitable for many lipid-based formulation approaches. For “brick dust” molecules, the main strategies used tend to focus on crystal modification, including salt formation and cocrystals.
A further approach is to enhance drug dissolution kinetics. Drug solubility is an equilibrium measure; the rate at which solid drug, or drug in a formulation, passes into solution (i.e., the dissolution rate) is also a critically important parameter. Because intestinal transit time is relatively rapid, a drug with a very slow dissolution rate may not have sufficient time to dissolve in the GI fluids for absorption to take place. Increasing the dissolution kinetics can therefore result in an improved bioavailability for oral formulations. Mechanisms of improving drug dissolution include reducing particle size (e.g., NanoCrystals®), selecting a metastable polymorphic form, and using amorphous solid dispersions (ASDs).
All these approaches are described in this chapter, beginning with a discussion on particle-size reduction technologies.
According to the Noyes–Whitney equation (Chapter 2, Equation 2.1), the dissolution rate of drug particles is proportional to the surface area of the particles in contact with the dissolution medium. A decrease in drug crystal size results in an increased surface area to volume ratio (Figure 3.2); therefore, reducing the crystal size of a drug powder will increase its dissolution rate. For example, if the crystal size is reduced from 1 μm to 100 nm, the surface area increases 10-fold, which should lead to a 10-fold enhancement of the dissolution rate. For drugs where dissolution is the rate-limiting step, improved dissolution in vivo will translate to higher, and more uniform, bioavailability. Furthermore, as described by the Prandtl equation, the diffusion layer thickness around the drug crystal may also be decreased, thus resulting in an even faster dissolution rate.
FIGURE 3.2 Mechanisms to increase solubility/dissolution rate by nanocrystal formation.
The solubility per se also increases as a result of reducing crystal size. Assuming that a particle is spherical, dependence of solubility on particle size can be described by the Ostwald–Freundlich equation:
where
C(r) and C(∞) are the solubilities of a particle of radius r and of infinite size
γ, M, and ρ are the interfacial tension at the particle surface, the molecular weight of the solute, and the density of the particle, respectively
According to this equation, solubility increases with a decrease in particle size, i.e., an increase in surface curvature. However, an example of the calculation in Figure 3.2, in which typical values are substituted for Equation 3.1, shows that an increase in solubility is almost negligible for crystals of 100 nm (1.02-fold) and only 1.27-fold at 10 nm. A crystal size of 10 nm cannot be produced with current formulation technologies. Additionally, the API crystal may not be spherical, whereas this effect is only valid when an increase in surface curvature is achieved.
Nanocrystals produced by top-down technologies (see Section 3.2.2.1), should also expose high-energy surfaces to the outer environment. This further enhances the dissolution rate (Figure 3.2). This mechanism is not applicable for nanocrystals produced by bottom-up procedures.
An increase in the surface area is thus likely to be the dominant mechanism for the enhanced dissolution in most cases. Micronization, the process of reducing drug crystal to the micron size range via milling techniques, has long been used in the pharmaceutical industry as a means of improving drug formulation and oral bioavailability. Recent improvements in the technology now allows for size reductions to extend even further: “nanosizing” refers to API crystal size reduction down to the nanometer range, typically ca. 100–300 nm, thereby providing a considerable increase in surface area and thus dissolution rate. “Nanomaterials” are defined by the FDA as materials with a length scale of approximately one to one hundred nanometers in any dimension. Although current commercial nanosized preparations typically have a size range of 100–300 nm and are thus outside this range, exceptions are also accepted if the material exhibits dimension-dependent properties or phenomena.
In this chapter, the authors use the term “nanocrystal” in the general sense, to describe any drug crystal in the nanometer size range, whereas NanoCrystals® denote a patented technology of Elan Corporation, described in Section 3.2.2.1. Once a drug is nanosized, the nanocrystals can be formulated into various dosage forms, including injectables, tablets, capsules, and powders for inhalation; they are thus suitable for delivery via a wide variety of routes. Dispersions of nanocrystals liquid media are known as “nanosuspensions.” Nanosuspensions prepared in water can be used as granulation fluids for the preparation of tablets; produced in oils, they can be used directly to fill capsules. Nanosuspensions may be stored in the liquid form, but postpreparation workup is also possible, such as spray-drying and freeze-drying, to obtain nanocrystals in a dry powder form. Sugars may also be added to formulations, to function as protectants during the drying process.
Nanosized formulations on the market include Avinza® (morphine sulfate), Focalin® XR (dexmethylphenidate hydrochloride), Megace ES® (megestrol acetate), Ritalin® LA (methylphenidate hydrochloride), Tricor® (fenofibrate), Triglide® (fenofibrate), and Zanaflex Capsules® (tizanidine hydrochloride).
Since nanocrystals have high-energy surfaces, stabilizers are needed to prevent irreversible aggregation. Steric stabilization can be achieved by the adsorption of hydrophilic polymers and/or surfactants onto the particle surface. Common polymers and/or surfactants used to provide steric stabilization include poly(vinylpyrrolidone) (PVP), hydroxypropyl methylcellulose (HPMC), hydroxypropylcellulose (HPC), a-tocopheryl PEG-1000-succinate (TPGS), polysorbate (Tween 80), and the pluronic surfactants F68 and F127. The repulsive steric layer prevents the particles from approaching each other. However, in most cases, the use of steric stabilization alone is not sufficient for nanosuspension stability. Further stability can be conferred by the adsorption of charged molecules, such as ionic surfactants (sodium lauryl sulfate and docusate sodium), onto the particle surface. In this case, electrostatic charge repulsion provides an electrostatic potential barrier to particle aggregation. Surfactants also help in the wetting and dispersion of the drug particles, which are usually very hydrophobic. Nanocrystals may have advantages over other surfactant-containing formulations such as emulsions and micelles, because the level of surfactant required is much lower, being only the amount necessary to stabilize the solid–fluid interface.
“Top-down” technologies involve disintegration methods, i.e., starting with coarse crystals and applying forces to reduce them to the nanocrystal range. As stated earlier, these top-down technologies expose high-energy surfaces to the outer environment, which further enhances the dissolution rate (Figure 3.2). The two most widely used technologies are media milling and homogenization.
Media milling. A milling chamber is initially charged with milling media: tiny balls, typically 1 mm or less, comprising materials such as ultradense ceramic media, or glass beads. A suspension of the API, with appropriate stabilizing agents, is then added to the chamber, and the chamber is rotated at a very high shear rate under a controlled temperature. The forces generated from the impaction of milling media with the drug cause crystal disintegration to the nanosize range. Processing time, as well as other operational parameters (milling speed, media load, media size, temperature, additives, etc.) can be tailored in order to maximize the crystal-size reduction process for each particular API. The process can produce stable, nanosized dispersions, with very tight, monodisperse, crystal-size distribution profiles. However, this method carries the risk of media ball erosion, which could result in the presence of unwanted media residues in the final product. For this reason, high-abrasion-resistance balls are used, and the final nanosuspension must be analyzed to ensure the absence of trace impurities.
NanoCrystals® are a proprietary wet milling technology from the Elan Corporation, which uses a highly crossed-linked polystyrene resin (PollyMill®) as the milling media (Merisko-Liversidge and Liversidge 2011). A crude slurry of the poorly water-soluble API, in a water-based stabilizer solution, is then added and subjected to shear forces. The NanoCrystal® particles of the drug are stabilized against agglomeration by the surface adsorption of patented, generally regarded as safe (GRAS), stabilizers.
The NanoCrystal® technology is used in a variety of commercially available preparations, where reformulation of the poorly soluble drug has resulted in many advantages. Sirolimus is a water-insoluble immunosuppressant drug, which was originally marketed as a self-emulsifying oral solution that required refrigeration and necessitated a complicated reconstitution procedure. In contrast, the NanoCrystal® tablet formulation (Rapamune®) offers improved bioavailability, less fluctuations in blood levels, easier storage (as no refrigeration is required), and improved palatability. Another example is the new chemical entity MK-0869, which was developed to treat chemotherapy-induced nausea and vomiting. The NanoCrystal® formulation (Emend®) resulted in a 600% improvement in bioavailability. Furthermore, in contrast to the original formulation, there is no need to take the drug with food (an important issue for this patient group, who are suffering from nausea and vomiting). Invega Sustenna® is the first commercial depot formulation product using NanoCrystal® technology, for the delivery of paliperidone palmitate in the management of schizophrenia.
High-pressure homogenization. This process involves the application of high shear and impaction forces to drug suspensions in order to reduce their particle size to the nanoscale. A number of commercial technologies now exist, including SkyePharma’s Dissocubes®, which can produce stable nanoparticle suspensions in water at room temperature. Triglide® (fenofibrate) is manufactured using this technology. The Nanopure® technology (PharmaSol GmbH) enables the production of nanosuspensions in nonaqueous media, for example with oils and PEG.
3.2.2.2 Bottom-Up Technologies
Nanocrystals may also be produced via “bottom-up” technologies, which involve assembly methods, i.e., starting from molecules in solution, then building up to form solid nanocrystals. Note that bottom-up procedures may produce metastable crystalline forms, including the amorphous state; the advantages and disadvantages of which are discussed in Section 3.6. Supercritical fluid technologies are being studied, although the technology is at an early stage. More developed methods include (1) precipitation and (2) emulsion as template.
Precipitation. Typically, the water-insoluble API is dissolved in an organic solvent, which is then mixed with an antisolvent, usually water. The addition of water causes a rapid supersaturation (nuclei formation) and growth of nanosized crystalline or amorphous drug. The limitation of this technique is that the drug needs to be soluble in at least one solvent, and the solvent needs to be miscible with the nonsolvent. This technology is available from DowPharma and BASF Pharma Solutions.
Emulsions as template. An emulsion is initially prepared comprising an organic solvent, or a mixture of solvents, loaded with the drug, which is dispersed in an aqueous phase containing suitable surfactants. The organic phase is then evaporated so the drug particles precipitate instantaneously to form a nanosuspension stabilized by surfactants. It is possible to control the particle size of the nanosuspension by controlling the size of the emulsion droplets. However, the possible use of hazardous solvents in this process raises safety and cost concerns.
A hybrid approach is also feasible: for example, Baxter’s Nanoedge® technology employs both “bottom-up” and “top-down” approaches, through microprecipitation and also homogenization. Significant progress in nanocrystal preparation technology is being made, which should lead to substantially more products being brought to market in the near future.
Salt formation, as described in Chapter 2 (Section 2.2.1), is the most common and effective method of increasing solubility and dissolution rates of acidic and basic drugs. In fact, more than 50% of the drugs currently listed in the USP are salt forms. The actual solubility of a salt, which is governed by the solubility products of the API and the counter salt, may not be much better than that of the free form. However, the dissolution rate
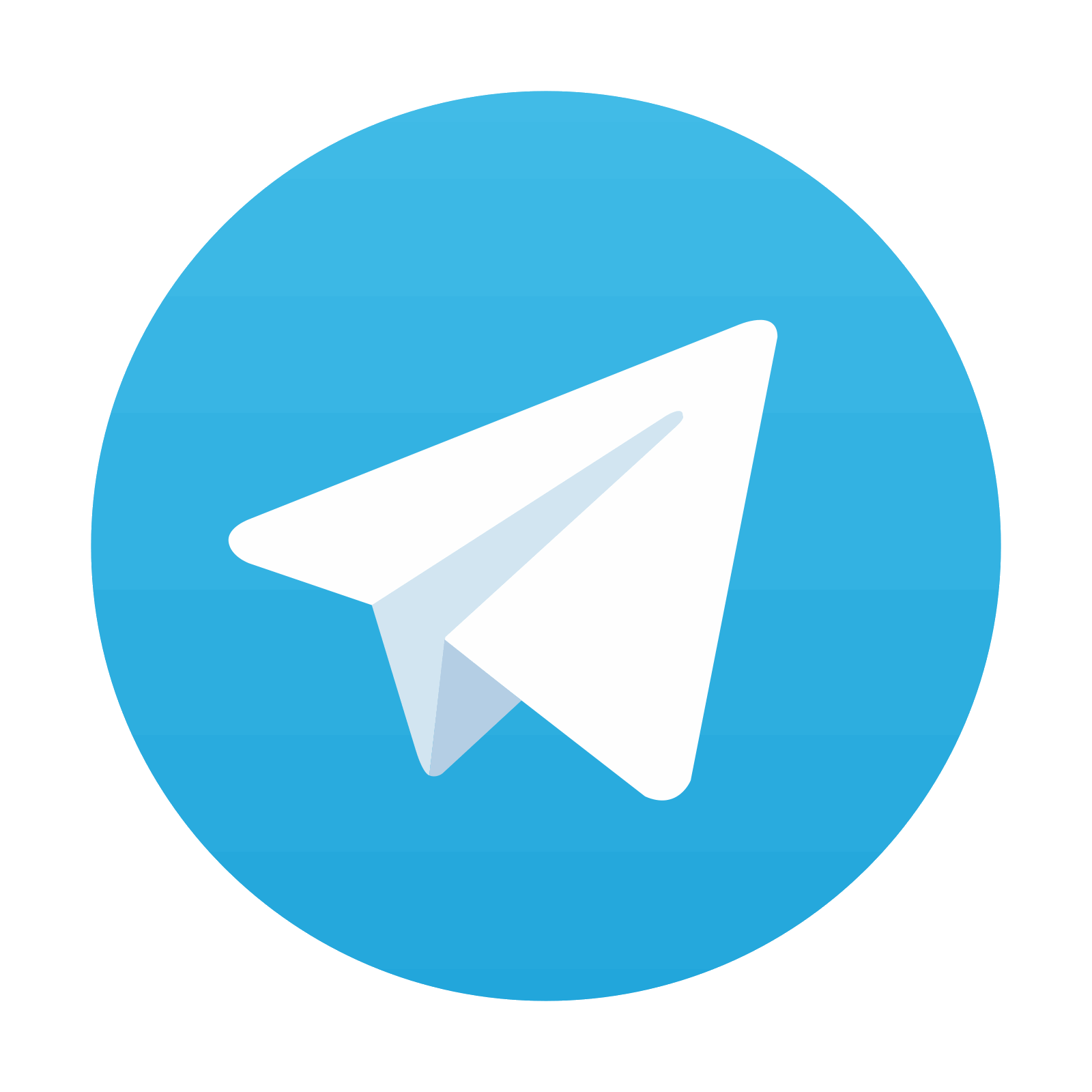
Stay updated, free articles. Join our Telegram channel
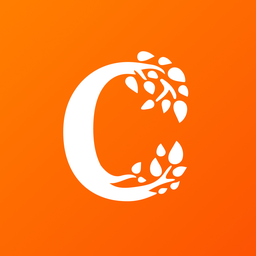
Full access? Get Clinical Tree
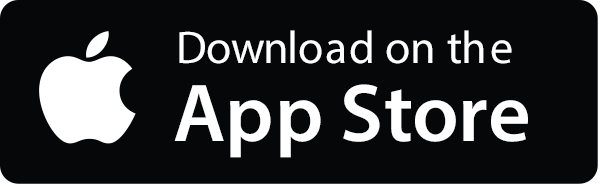
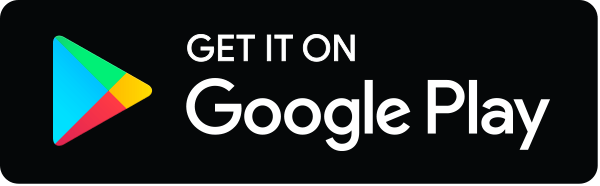