Implantation and Placenta
Introduction
Interest in placental examination has increased in the last two decades, with more appreciation by obstetricians and neonatologists of the contribution the report can make to understanding adverse perinatal outcomes. Not every placenta need be examined, and a triage system is essential. Indications for examination are essentially any disease of the mother, and abnormality of pregnancy, labor, delivery, or the immediate postnatal period. Placental abnormalities should prompt at least macroscopic evaluation. In most institutions, this constitutes 10–15% of deliveries. Placental examination is an integral part of the fetal or perinatal autopsy and adds conclusive or important information in between one-third and two-thirds of such cases.1,2 While the delivery suite may be the ideal place to initiate microbiology and cytogenetic testing, full pathologic evaluation requires a laboratory-based grossing station with adequate photographic and other facilities. For those cases judged by the pathologist to only require gross examination, retention of formalin-fixed tissue can permit subsequent histologic examination in cases where an abnormality becomes apparent in neonatal life, rather than at birth. In addition to providing information to clinicians and parents, the placenta has been described as ‘an amazingly good defensive witness in “bad baby” lawsuits.’3
Anatomy and Embryology
Implantation and Early Pregnancy
The process of implantation has three phases: muscular, adhesive, and invasive. The muscular phase concerns transport of the conceptus to the optimal site for implantation, which in humans is the mid to high posterior wall of the uterus in the mid-sagittal plane (Figure 32.1). Implantation in different locations may be associated with different pathologies, e.g., fundal placentation shows an association with intrauterine growth retardation (IUGR).4 During the adhesive phase, the normally repulsive interactions between two epithelial surfaces (endometrium and trophoblast) are reversed. In the invasive phase, irregular projections of syncytiotrophoblast invade into the endometrium (Figure 32.2). This phase lasts until approximately day 8 post conception and is called the prelacunar phase, based on the appearance of the blastocyst. At day 8 vacuoles appear in the syncytiotrophoblast, which become confluent to form lacunae. This change commences at the implantation pole and becomes confluent over the blastocyst by day 13. At this stage, the earliest forms of chorionic villi begin to form (Figure 32.3) and the primary chorionic plate consists of a continuous layer of cytotrophoblast on the embryoblast side of the lacunae. Infiltration of the pillars of syncytiotrophoblast surrounding the lacunae by cytotrophoblast from the chorionic plate is followed by expansion of these pillars as extraembryonic mesenchyme follows cytotrophoblast. The outermost layer of trophoblast (the trophoblast shell) is formed by syncytiotrophoblast, and later by cytotrophoblast as well. Cytotrophoblast continues to invade endometrium and is seen as clusters of extratrophoblast (‘X’ cells) and trophoblastic giant cells in what will become the basal plate. The lacunar space becomes the intervillous space, and the embryologic development of villi proceeds during gestation. These developments are maximal at the deep aspect of the blastocyst and normally only this persists to form the true placenta (chorion frondosum). The remainder atrophies (chorion laeve) (Figure 32.4).
Figure 32.2 Primitive trophoblast. Chorionic villi have not yet formed in the pregnancy of about 1 week’s duration.
Figure 32.3 (A) Day 14 conceptus as a tiny implant in superficial endometrium. (B) Detail of the earliest stage of chorionic villous formation.
Figure 32.4 Normal membrane in a third trimester placenta. (A) Amnion (A), parts of which include amnionic epithelium (AE), amnionic mesoderm (AM), and spongy level (S); the chorionic plate (C) is composed of chorionic mesoderm (CM) and trophoblast (T); beneath that is the maternal decidua (D). (B) Both the trophoblast and amnionic epithelium are markedly reactive for cytokeratin (CAM 5.2).
The decidua is not merely the passive recipient of the conceptus, but plays an active role in successful placentation.5 Cytotrophoblastic cells stream out from the tips of the anchoring villi, penetrate the trophoblastic shell, and colonize the decidua and adjacent myometrium of the placental bed. These cells, which are reactive for human placental lactogen and cytokeratins, are called interstitial extravillous trophoblast. A subset of these called ‘intravascular extravillous cytotrophoblast’ invades and plugs the lumens of the decidual spiral arteries. The cells destroy the endothelium and the elastic and muscular tissues of the media, which are then replaced by fibrinoid material derived from fibrin and trophoblastic secretions. This produces large diameter vessels lacking intrinsic tone that allow a high-flow, low-pressure system to develop. Low oxygen tension may help control entry of cytotrophoblast into the S-phase of the cell cycle, while proliferation and high ambient oxygen tension may lead to an invasive phenotype. The higher oxygen tensions in the nontransformed spiral arteries may induce the expression of invasive integrins, a vascular adhesion molecule phenotype, and cessation of mitotic activity. This may explain why trophoblast only superficially invades the uterine veins. Trophoblast may switch to a noninvasive phenotype by becoming multinucleate.
Development in the first trimester takes place in a relatively hypoxic environment, and this is protective to the embryo. A trophoblast plug prevents maternal blood entering the intervillous space and early embryonic nutrition is provided by endometrial glands, perhaps facilitated by endoglandular trophoblast.6 The trophoblast plug is seen to be deficient in many early losses (Figure 32.5). This results in the precocious onset of the maternal circulation, exposing the developing placenta to a higher oxygen concentration and to a higher arterial pressure. As spiral artery transformation is less effective peripherally, oxidative stress may be the mechanism by which villous regression occurs in the chorion laeve.7 Patterns of regression influence placental shape and cord insertion, and localized abnormalities of flow may result in a cord that was initially paracentral becoming marginal or velamentous. Noncentral cords are associated with lower birth weights, suggesting that their vasculature is less metabolically effective.8 Suboptimal or shallow implantation results in inadequate conversion of maternal spiral arterioles in the inner third of the myometrium. The resultant retention of vascular smooth muscle permits intermittent pulsatile contraction, resulting in mechanical and oxidative stress to the developing placenta.
Figure 32.5 A transforming spiral artery. Intravascular trophoblast and extravillous interstitial trophoblast are present.
Placental growth trajectories are established by the end of the first trimester, with cases of IUGR having smaller placentas than normal at 12 weeks, but similar growth after that. Villous development shows a major change from growth to differentiation at the end of the second trimester. Protrusions of trophoblast (trophoblast sprouts) into the lacunae are the forerunners of villi. Initially, the sprouts consist of syncytiotrophoblast, which are followed by cytotrophoblast and by connective tissue containing fetal capillaries. The villi thus formed are termed mesenchymal villi and are the precursors of all other villous types. While they are the dominant type in the first trimester, some trophoblast sprouting and mesenchymal villous development probably occurs up to term.
Immature intermediate villi (100–200 µm in size) are formed from mesenchymal villi and are primarily responsible for placental growth (Figure 32.6). They have a complete trophoblastic mantle with many cytotrophoblastic cells present, but lack vasculosyncytial membranes. The syncytial nuclei are evenly dispersed without knots. They have a loose (reticular) stroma with abundant stromal channels containing Hofbauer cells. They are the dominant villous type seen in the second trimester and are transformed into stem villi when their production from mesenchymal villi decreases.
Mature intermediate villi (Figure 32.7) are formed from the mesenchymal villi in the third trimester and give rise to the majority of terminal villi. They are 60–150 µm in diameter and contain capillaries, arterioles, and venules. The stroma is loose and the vessels constitute less than half the villous cross-sectional area. The syncytiotrophoblast has a uniform structure without vasculosyncytial membranes or knots. At term, mature intermediate villi make up one-fourth of the parenchyma.
Terminal villi (40–60 µm) (Figure 32.8) are the site of gaseous exchange and derive from the mature intermediate villi. They arise as capillary growths which, in exceeding that of parent mature intermediate villi, result in trophoblastic protrusions. The capillaries are dilated and constitute over 50% of the villus cross-sectional area. Optimal gas exchange requires the formation of vasculosyncytial membranes. The syncytiotrophoblast nuclei become pushed to one side so that only an attenuated layer of syncytiotrophoblastic cytoplasm remains applied to its basement membrane, which is itself applied to the capillary’s basement membrane. The capillaries are 3–5 mm long and each maintains its own nonbranching structure. They do not form a capillary network, but remain as long capillaries. In addition, the capillaries have varicosities where their diameters enlarge considerably. The exact functional significance of these varicosities is unknown, although a regulatory function where vascular deformation alters flow has been proposed. These villi appear at 27 weeks of gestation and increase in number until term, when they make up 40% of villi.
Examination of the Placenta
Umbilical Cord
Abnormalities of the cord are associated with adverse outcomes and with stasis-induced abnormalities in the fetal vasculature.9 Cord length should be measured, but the possibility that sections of the cord may have been removed shortly after delivery should be excluded before a short cord is reported. The average cord length at term is 60 cm. Longer cords are associated with hypermotility and shorter ones with hypomotility. Neural tube defects and chromosomal abnormalities are sometimes the underlying cause of the latter. The lower limit for a normal cord varies, but seems to be around 35 cm and a short cord is associated with an increased risk of death in term infants. In an 18 year retrospective review, excessively long cords (≥70 cm) were associated with a range of gross and microscopic features, and with abnormal neurologic status in infants.10 Clinical or pathologic abnormalities of the cord were found in 70% of infants with fetal thrombotic vasculopathy.11 False knots (vascular loops) may be present (Figure 32.9), but usually are of no clinical significance. The maximum diameter should be measured both, sonographically12 and pathologically; a thin cord (<8 mm) is associated with poor flow and growth restriction.
True knots (Figure 32.10) occur in approximately 1% of pregnancies. They are more likely to occur with a long cord, with polyhydramnios, and with male fetuses.13 The knots may be single or multiple. Chronic changes (grooving of the cord, edema, vascular congestion, or thrombosis) or their absence should be specifically commented on. The fetus with a long cord is less able to exert traction on the knot in utero. The effects of a knot are mediated by its tightening with traction, causing vascular compromise. This may happen at delivery, but then the chronic changes will be absent. Documentation of a true knot in utero is difficult, and a loose knot may be formed after intrauterine death. The lack of a difference in blood gas values between neonates with true knots and those without supports the interpretation that most knots are clinically insignificant.14 However, a true knot is more commonly associated with other cord problems including nuchal cord and cord prolapse, either of which may contribute to the observed increase in antepartum (but not intrapartum) stillbirths. Cord pathology is associated with fetal thrombotic vasculopathy. A feature to be sought in such cases is an increase in nucleated red blood cells, a finding that supports a conclusion of subacute hypoxic stress.15
Coiling of the cord is normal. It is greater at the fetal end and is variable throughout the length of the cord. A literature review has indicated 0.17 ± 0.009 spirals completed per centimeter.16 The 10th–90th percentile range is one per 3 cm to one per 14 cm on this basis. The mean coiling index (number of coils/length in cm) has varied from 0.13 to 0.28:17 the ‘mean of the means’ of the studies listed was 0.20. A long and/or hypercoiled cord (Figure 32.11) will require a greater pressure gradient because of increased shear stresses. Both hypo- and hypercoiling of the cord are associated with a range of adverse pregnancy outcomes, including pregnancy loss and IUGR. The cord may show a stricture (Figure 32.12). Stricture and/or hypercoiling was reported in 19% of fetal deaths18 and thrombosis of chorionic plate vessels was seen in 54% of these cases. Given the importance of the diagnosis, a thoughtful review of coiling has called for ‘a return to basic but critical mensuration.’17
The cord may insert centrally, eccentrically, and marginally or have a velamentous insertion. The last two are the most significant. In marginal insertion, the cord joins the disc at its edge. Cord insertion directly onto the membranes (velamentous insertion) (Figure 32.13) occurs in approximately 1% of singleton deliveries. The vessels are at risk of compression and thrombosis, and rupture may lead to significant fetal blood loss and hypoxia. In addition to intrapartum events, velamentous insertion is associated with an increased risk of preterm delivery, low birth weight, and abnormalities of fetal heart rate.19 Vessels may be present in the membranes in up to 7% of placentas, but vasa previa (vessels in the membranes in advance of the presenting part) are less common (1 : 2500 deliveries). In addition to velamentous insertion, other risk factors for vasa previa include a bilobed or succenturiate placenta, a low-lying placenta, and multiple and IVF pregnancies. Prenatal diagnosis reduces the mortality rate, and screening of all twin pregnancies has been reported to be cost-effective.20 When examining a placenta where this has been queried clinically, a measurement of the length of the vessels in the membranes and a comment on whether they are intact or not is appropriate. We have found that marking a torn area with ink helps in microscopic evaluation.
In the third stage of labor, avulsion of the cord may occur due to traction and this may cause subamniotic hemorrhage. However, this form of hemorrhage may also occur where a central or eccentric insertion is furcate (Figure 32.14), i.e., where cord vessels have lost their cover of Wharton’s jelly and have splayed out prior to inserting into the disc. In most cases fresh subamniotic hemorrhage is of no clinical significance. In some cases there may be hematomas of the cord without apparent explanation (Figure 32.15).
The normal cord has three vessels—two umbilical arteries and one umbilical vein. A single umbilical artery, i.e., a two-vessel cord, occurs in 0.5–1% of deliveries (Figure 32.16). As the arteries may fuse in the 5 cm before insertion into the placenta, further sections nearer the fetal end of the cord should be examined before a single artery is reported. One-third of cases of single umbilical artery are associated with other congenital abnormalities, including trisomy 18, and abnormalities of the heart, gastrointestinal and urinary tracts, and central nervous system. Isolated single umbilical artery is associated in some studies with increased preterm deliveries, growth restriction, and adverse outcomes.21 Other studies did not show a difference in perinatal outcome or long-term development.22
Supernumerary vessels are rare and may be either arterial or venous. Tumors of the cord are also rare. Most are hemangiomas (Figure 32.17) but occasional angiomyxomas and teratomas have been reported. The muscularis of cord vessels may be focally thin. Confirmation of a possible association with congenital malformations is needed, given the small numbers of cases reported.23 Grossly or sonographically visible cysts may develop from the vestigial remnants that are usually found as incidental microscopic findings. These remnants may be of the allantoic duct (possessing a flattened or transition cell-type epithelium; Figure 32.18A) or omphalomesenteric duct (cuboidal epithelium with mucinous component; Figure 32.18B).
Figure 32.18 (A, B) Normal findings in umbilical cord. (A) Allantoic duct (possessing a flattened or transition cell-type epithelium), residual urinary tract system. (B) Omphalomesenteric duct (cuboidal epithelium with mucinous component), residual digestive system.
Membranes
Acute chorioamnionitis is mainly caused by bacteria. It is the major cause of preterm birth, but it is still not clear if it is a cause or effect of either preterm rupture of membranes or preterm labor.24 While there are clinical guidelines for recognition of infected membranes histologic chorioamnionitis is a better surrogate of intra-amniotic infection. The severity of chorioamnionitis shows a direct correlation with proteomic signatures of inflammation.25 Organisms may or may not be cultured: group B Streptococcus and Escherichia coli are important causes of chorioamnionitis, with organisms such as Ureaplasma urealyticum frequently seen in mixed culture.
A section of membranes should be taken, extending from the rupture site to the margin of the disc and prepared for histologic examination as a roll. The yield is influenced by the extent of sampling, with increased yield up to four sections.26 Criteria for categorization of the maternal and fetal inflammatory responses as early, intermediate, or advanced, and grading of both as mild/moderate or severe have been published27 (Figures 32.19 and 32.20). These are summarized in Table 32.1. Severity of the maternal response is defined by the presence of subchorionic microabscesses, or three or more confluent polymorph bands, and in the fetal response as near-confluent bands. Amnionic inflammation is a better predictor of neonatal sepsis than funisitis in preterm gestations.28 The inflammatory pattern and the time taken to mount an inflammatory response may primarily reflect the virulence of the infecting organism. Organisms of low virulence (e.g., Ureaplasma, Mycoplasma, and anaerobes) may cause preterm labor, but not bacteremia in the neonate. They probably take days rather than hours to reach the placenta. Virulent organisms, e.g., group B Streptococcus, E. coli, and Listeria, can cause bacteremia and may reach the placenta in hours rather than days. However, membrane integrity, maternal immune status, and cytokine gene polymorphisms play a role. Gestational age is also of importance; chorionic vasculitis is less prevalent with increased gestational age. Data correlating chorioamnionitis with bacterial in situ hybridization suggest that organisms invade through a focal area of the membranes, proliferate in the amniotic fluid, and invade the membranes from there.29 The presence of acutely inflamed membranes should prompt a search for associated lesions, such as thrombi, and exclusion of coexistent pathology, such as retroplacental hemorrhage, where chorioamnionitis is more common.
Table 32.1
Categorization of Inflammatory Responses in Acute Chorioamnionitis
Response | Stage | Features |
Maternal | 1 | PMN in subchorionic fibrin or membrane trophoblast |
2 | PMN in fibrous chorion and/or amnion | |
3 | PMN karyorrhexis, necrosis of amnion | |
Fetal | 1 | PMN in umbilical vein or chorionic plate vessels |
2 | PMN in one or more umbilical arteries | |
3 | PMN ± debris with perivasculitis |
PMN, polymorphonuclear leukocytes.
Adapted from Redline et al.27
Figure 32.19 Acute chorioamnionitis, stage 1 (Table 32.1). The inflammation involves the trophoblast, but not the spongy layer of the amnion.
Figure 32.20 Acute chorioamnionitis, stage 3 (Table 32.1). The inflammation pervades all structures and destroys the amnionic epithelium.
In addition to bacteria, fungi and viruses may also cause infection via the ascending pathway. In Candida chorioamnionitis, numerous yellow-white spongy flecks stud the membranes and umbilical cord (Figure 32.21).
Figure 32.21 (A, B) Candida funisitis. (A) Small white flecks are on the surface, which (B) microscopically are Candida organisms (arrows).
The clinical significance of chorioamnionitis and funisitis depends on gestational age and severity. Ascending infection is a major cause of preterm premature rupture of the membranes, which accounts for 30–40% of preterm births. Increasing stages of chorioamnionitis show a significant association with funisitis, preterm birth, and perinatal death. Funisitis has been directly linked with development of fetal germinal matrix hemorrhages, choroiditis with intraventricular hemorrhage, and periventricular leukomalacia.30,31 For term infants, there is an increased risk of sepsis following chorioamnionitis, but neurologic morbidity may be related to other complications of labor.32 In one study of very low birth weight infants, vascular thrombi associated with chorioamnionitis were felt to account for neurologic impairment.33 An association between chorioamnionitis and adverse developmental outcome has not been demonstrated in other studies on similar groups.34 Defining initiators of preterm birth (i.e., uteroplacental insufficiency vs inflammation) may be important in documenting their long-term effects35 and placental inflammation shows an association with poor neonatal growth.36
Decidual necrosis manifests as a shaggy, cream-yellow area, usually present near the margin of the placental disc. Nodularity of the amnion may be due to either amnion nodosum or squamous metaplasia. Amnion nodosum is a consequence of oligohydramnios and presents as 1–3 mm nodules that may be relatively easily detached. Microscopy shows aggregates of amorphous and cellular debris (Figure 32.22), and may include detached hair. With squamous metaplasia, which has no known clinical associations, the nodules are only detached with difficulty.
Meconium on Cord and Membranes
Meconium-stained amniotic fluid is seen in 14% of deliveries, and is present in approximately 1% of deliveries at 32 weeks, compared with 100% at 42 weeks. Its passage at term may be physiologic, but is generally felt to reflect hypoxic stress in the fetus.37 We regard the presence of scanty macrophages with non-hemosiderin pigments as normal and physiologic: indeed, fetal defecation has been documented sonographically.38 More extensive meconium passage produces a green discoloration grossly. Meconium may reach superficial amnionic macrophages (Figure 32.23) in 1 hour and chorionic macrophages in 3 hours. They may remain in the amnion or chorion for a week after the meconium is no longer visible in the amniotic fluid. Meconium acts as a vasoconstrictor on the fetoplacental vasculature, and may result in necrosis of smooth muscle cells of umbilical cord vessels (Figure 32.24) or cause ulceration of the cord.39 The changes in muscle cells may mimic a vasculitis. The inflammation that meconium induces is maximal in the cord, but is less intense and more focal than that due to the vasculitis of chorioamnionitis. Documentation of its presence is important, as thick meconium is associated with adverse pregnancy outcome. If aspirated, meconium produces significant pneumonitis.
Figure 32.23 Meconium in a macrophage (arrow) is a light brown pigment usually less refractile than hemosiderin.
Architectural and Developmental Abnormalities
The circummarginate form (Figure 32.25) has no known clinical associations. The circumvallate form (Figure 32.26), however, is associated with threatened abortion, membrane rupture, and antepartum hemorrhage leading to prematurity, but not to an increase in perinatal mortality. Circumvallation is significantly associated with iron-laden macrophages in the membranes, termed ‘diffuse chorioamnionic hemosiderosism,’40 suggesting that circumvallation may be caused by chronic peripheral separation of the placenta. Both types of extrachorial placentation are frequently partial and mixtures between the two and normal placentation are more frequently encountered.
Figure 32.26 Circumvallate placenta. The membranes fold back upon themselves on the surface of the placenta.
A bilobed placenta has two nearly equal sized discs with the umbilical cord inserting between the two, either in a velamentous fashion or marginally on the larger disc. As discussed previously, inadequate blood flow leads to trophotropism and placentation on both the anterior and posterior endometrial surfaces. These placentas are associated with multiparity, older maternal age, previous history of infertility, assisted reproduction, retention, and abnormal adherence. The vessels between the two may thrombose or present as vasa previa. Higher orders of lobation are extremely rare.
Accessory (succenturiate) lobes are found in 6% of placentas and are areas of placental tissue joined by either an isthmus or velamentous vessels to the main disc (Figure 32.27). If symptomatic, they may present as placenta previa with or without fetal hemorrhage or be retained in utero postpartum. Placenta membranacea arises when there is failure of villous regression to form a chorion laeve. Thus, an abnormally thin placenta comes to cover an unusually large area of the uterine lining. The entire conceptual sac is covered with villi and the placenta becomes, in addition, a placenta previa. The placenta on cut section is thinned from the normal 2.5 cm or 3 cm to perhaps 1 cm or less in the fixed state. This condition is sometimes associated with mid-trimester antepartum hemorrhage. It may also sometimes show undue adherence, but normal third-stage delivery usually occurs.
< div class='tao-gold-member'>
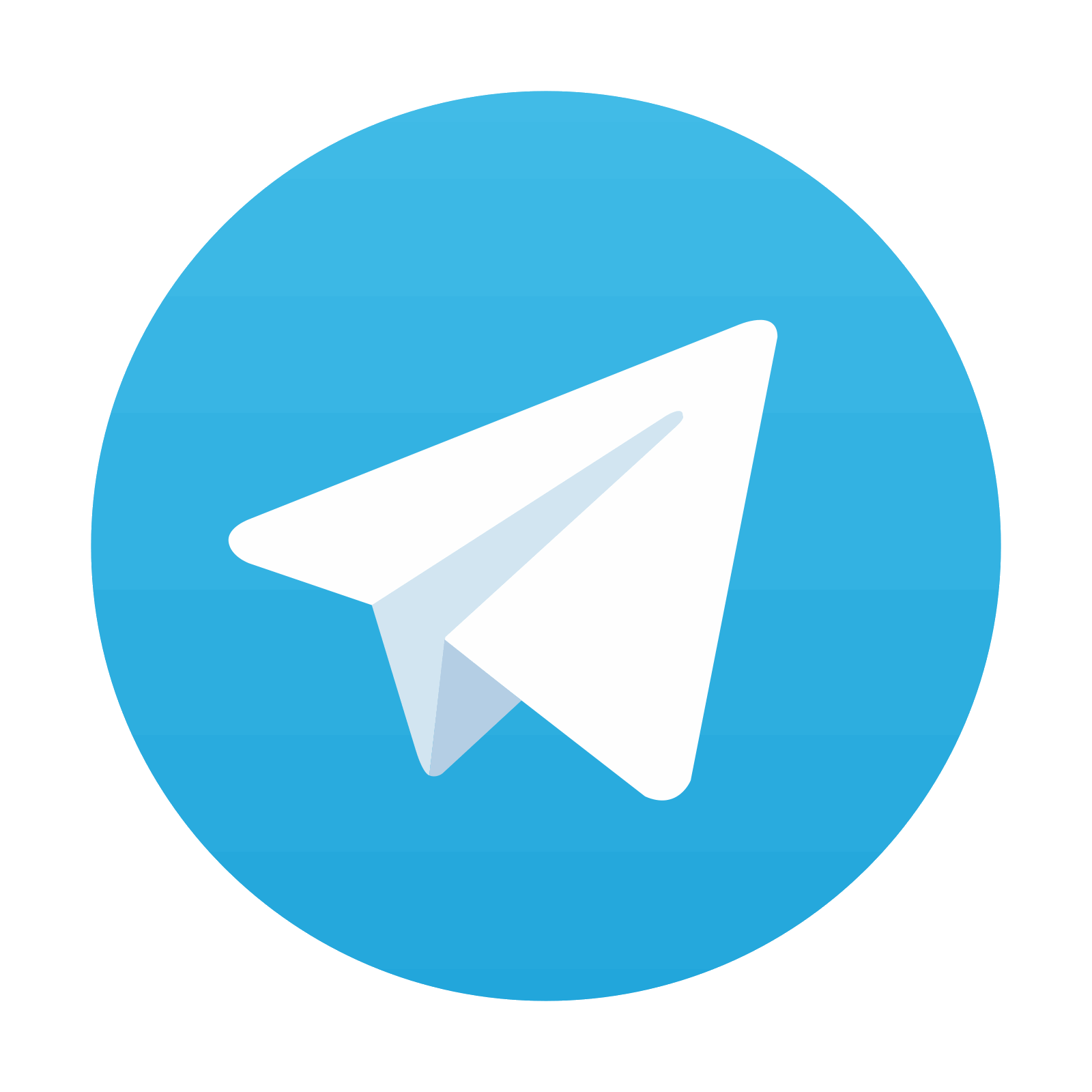
Stay updated, free articles. Join our Telegram channel
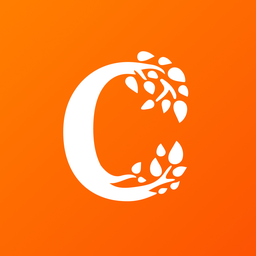
Full access? Get Clinical Tree
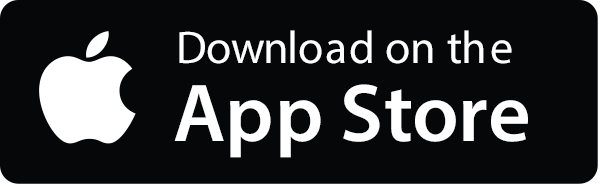
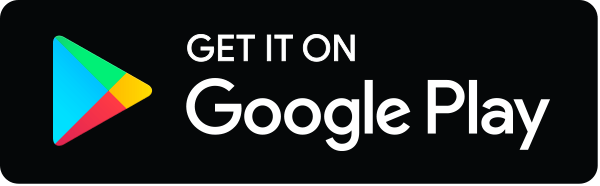