CONTENTS
14.2.1 Hydrogels Based on Biopolymers
14.2.2 Synthetic Polymerization
14.2.2.1 Step-Growth Polymerization
14.2.2.2 Chain-Growth Polymerization
14.2.3 Structural Characteristics of Linear Polymers
14.2.4 Chemically Cross-Linked Networks
14.2.5 Physically Cross-Linked Networks
14.3 Fundamentals of Partitioning, Permeability, and Solute-Release Properties of Hydrogels
14.4 Stimulus-Sensitive Hydrogels
14.4.1 A Balance of Forces Determines the Degree of Swelling
14.4.2 Stimulus-Sensitive Hydrogels for Drug Release
14.4.2.1 Temperature-Sensitive Systems
14.4.2.3 Glucose-Sensitive Systems
14.4.2.6 External Stimulus-Sensitive Systems
14.4.2.7 Multiresponsive Systems
Hydrogels are polymer networks that contain a substantial amount of water. Dry polymer networks can absorb tens, hundreds, or even thousands of times their weight in water without dissolving, as illustrated in Figure 14.1. As a result, hydrogels have properties that are very similar to those of soft biological tissues such as cornea, cartilage, and intercellular matrix, and because of these characteristics, they are of great utility in pharmacy and in other biomedical fields. First, they are soft and slippery and have a very low interfacial tension when they are in contact with tissues. As a result, they often cause little irritation. Second, hydrogels can behave both like solids and like liquids. Like solids, they are elastic and return to their original shape after they are momentarily deformed. Like liquids, they permit diffusion of small molecules such as salts, metabolites, and drugs. Under proper circumstances, they also allow larger molecules such as proteins and nucleic acids to diffuse.
A hydrogel can be envisioned as a bulk of water through which polymer strands are suspended. As illustrated in Figure 14.2, these strands are connected together by cross-links, which provide elasticity and prevent the strands from coming apart. The distance between strands and the frequency of cross-linking, which determine the mesh size, can be controlled; by this means, the partitioning of molecules into the hydrogel and their rates of diffusion can be regulated. For this reason, hydrogels are often used in controlled-release drug delivery.
FIGURE 14.1 A hydrogel is a dry polymer network that is swollen with water but does not dissolve.
As elastic materials, hydrogels can exert forces against confining or attached structures. As will be described later, the degree of swelling and shrinking of hydrogels can be affected by physical and chemical stimuli. Thus, the hydrogels can act as “artificial muscles,” which direct the motion of such structures. This property has also been utilized in controlled-release applications.
Table 14.1 provides a list of present and potential future applications of hydrogels. Soft contact lenses were initially developed in the early 1960s as alternatives to hard contacts, which are irritating and can damage the cornea. While soft contact lenses may contain only about 40% water, this is enough to confer flexibility, slip, and compatibility with the underlying cornea. Because optical properties are of foremost importance, hydrogels in contact lenses must remain constant in their swelling during use. Other applications involving hydrogels with constant swelling are listed in bold in Table 14.1.
A tantalizing property of hydrogels is their ability to alter their swelling depending on an applied stimulus, as depicted in Figure 14.3. Commonly studied stimuli include changes in temperature, pH, and ionic strength, but other possible stimuli include the concentration of specific ions such as Ca2+ or glucose and other sugars, and the presence of specific antigens or DNA sequences, electric or magnetic fields, and even light. Applications involving “stimulus-sensitive” hydrogels are listed in italics in Table 14.1. (Note: some investigators refer to stimulus-sensitive hydrogels as “smart” or “intelligent.” We eschew such anthropomorphic terminology.)
TABLE 14.1
Present and Future Applications of Hydrogels
Present | Futurea |
Soft contact lenses | Burn, wound dressings |
Gel permeation chromatography | Catheter, suture coatings |
Ion exchangers | Fire retardants |
Gel electrophoresis | Stimulus-sensitive drug delivery |
Drug delivery (e.g., capsules, matrices) | (Bio)sensors |
Cosmetics | Microparticulate drug and gene carriers |
Food additives | Tissue culture, tissue engineering, and bioartificial organ supports |
Fragrances | “Active” separations |
Dessicants | Artificial muscle |
Toys | Switchable chromatography |
a Applications in which size and shape are expected to be constant in time are in bold, while applications in which the hydrogel changes size or shape in response to various stimuli are in italics. |
FIGURE 14.3 Stimuli that may affect hydrogel swelling.
This chapter serves as an introduction to hydrogels. More details can be found in the references listed at the end of the chapter. We first describe methods of synthesis. We then discuss factors that affect their swelling, elasticity, solute partitioning, and permeability properties. Finally, examples of present and future applications in the pharmaceutical arena are presented.
14.2.1 HYDROGELS BASED ON BIOPOLYMERS
“Natural” hydrogels are produced from biological polymers. Because they are the result of biological synthesis, the structure of biopolymers comprising the hydrogels is often well controlled. Their building blocks, by nature, are often biocompatible, although care must be taken to purify the biopolymers and remove antigens and pyrogens. Examples of biopolymers used to form natural hydrogels include fibrous proteins such as collagen, gelatin (denatured collagen), and fibrin and polysaccharides such as calcium alginate, agarose, hyaluronic acid, cellulose and its derivatives, dextrans, chitosan, carrageenans, pectins, and pullulans.
Long-chain biopolymers often form physical cross-links by winding two or more chains to form helical domains. Such cross-links can be loosened by melting or by applying chemical denaturants. Calcium alginate hydrogels are produced when alginic acid chains form coordination complexes that are wrapped around calcium ions, leading to a so-called “egg case” structure. When calcium is replaced with sodium ions, these cross-linking structures are dissolved and the alginate becomes fluid. Physical cross-linking can also occur by formation of microcrystalline domains, usually driven by hydrogen-bonding interactions, as in cellulose, or by aggregation of hydrophobic domains as in pullulans.
Physical cross-links are advantageous since they can often be formed and reversed by mild processing techniques. On the other hand, physical cross-links may not be permanent, and with persistent stress, physically cross-linked hydrogels may deform permanently. Hydrogels formed from fibrous proteins can form more permanent, stress-resistant structures if they are chemically cross-linked. Here, a linker molecule such as glutaraldehyde forms covalent bonds between side chains on separate protein molecules. Companies specializing in biochemicals sell a variety of such cross-linkers, which are also used for protein conjugation. Chemical cross-linking of polysaccharides is often accomplished by chemistries that have been developed for synthetic polymers, as will be described in the following texts. Alternatively, biopolymers can be cross-linked by exposure to radiation, which works by forming free radicals along different polymer chains, which find each other and recombine, forming covalent cross-links.
14.2.2 SYNTHETIC POLYMERIZATION
Synthetic polymers and their hydrogels are usually formed from petroleum-based organic chemicals. Except for some unusual cases, these systems exhibit less intrinsic structural regularity than biopolymers. Also, only a limited set of synthetic hydrogels are biocompatible. Nevertheless, synthetic hydrogels can be produced cheaply, and their chemical compositions and network structures are easily studied and controlled. In recent decades, there have been major advances in the synthesis of organic polymers, resulting in improved quality of hydrogels. Here, we will describe several methods for preparing hydrogels, beginning with a brief review of polymer synthesis.
14.2.2.1 Step-Growth Polymerization
In step-growth polymerization, polymer-building blocks or monomer units contain end groups that react with each other to form larger molecules having the same end groups. Imagine a monomer with two end groups, say A and B, that react with each other. Two monomers of structure A–B will form a dimer of structure A–B′–A′–B. The prime symbols in the middle indicate that the joined A and B units might be modified as a result of the reaction. For example, if A is an acid group and B is a hydroxyl group, then B′–A′ will be an ester linkage. If A is an acid group and B is an amide group, then B′–A′ will be an amide linkage. In these two examples, a water molecule is liberated in a condensation reaction. However, the dimer still retains unmodified A and B end groups, which can enter into reaction with other A–B monomers. Similarly, already formed chains with A and B end groups can react with each other to form even longer chains. Thus, reactions such as
are possible, for any chain lengths x and y. If the reactions are condensations, then the step-growth polymerization can also be called a condensation polymerization. The two reactions mentioned earlier lead, respectively, to polyesters and polyamides. It should be noted that A and B need not be distinct, provided that an end group can react with an identical end group. For example, two organic acids, –COOH, can combine to form an anhydride linkage, with a water molecule liberated. It is also possible to perform step-growth polymerizations from the initial monomers, A–A and B–B, since these can react to form A–A′–B′–B, which can then go on to form longer polymer chains.
Since condensation reactions are reversible, water removal is usually required for sustained growth. Also, step-grown reactions are particularly sensitive to impurities containing only one reactive end, which will cap the polymer and prevent further growth.
The step-growth mechanism just described will only form linear chains. As with biopolymers, such chains can be cross-linked through their side groups. However, an even simpler method exists. Along with the A-B monomers, a fraction of monomers with structure R-Af can be included, where R is a central group that is surrounded by f A groups, where f > 2. Similarly, other monomers of form R-Bf can be included. The R groups in these two monomers can be the same, but they need not be; nor do the fs, so long as they are both greater than 2. The fs are called functionalities. With these multifunctional monomers, the ends of growing linear chains can be linked together into networks, as diagrammed in Figure 14.4.
One characteristic of step-growth polymerization is that the individual polymer chains, or the chains connecting cross-links, are of variable length. The growth sequences of different chains vary, and the result is a distribution of chain lengths. Therefore, while the average chain length can be specified or determined experimentally, this variability must be regarded as a fundamental property.
14.2.2.2 Chain-Growth Polymerization
In chain-growth or addition polymerization, individual chains form by initiation at one end, where a reaction center is formed. Monomers are then added, one by one, during propagation steps, with the reactive center shifting to the last monomer added. Propagation proceeds until a termination event occurs, in which the growing chain reacts with a species that permanently blocks further monomer addition.
Free radical polymerization, the most common version of addition polymerization, is illustrated in Figure 14.5. In this class of reactions, the monomer units contain vinyl groups, denoted by ╕, which signifies a carbon double bond along with a (here unspecified) side group. When attacked by a free radical on one side, the double bond is converted to a single bond, and the free radical is shifted to the other side, where it is now available to attack a second monomer. This second monomer then attacks a third, and so on. Initiation of this chain reaction is brought about by thermal, photo, radiation, or redox decomposition of an initiator species, I, converting it to a free radical, which we denote by I·. The result is a long chain with the initiator at one end and a still reactive free radical on the other.
In most free radical polymerizations, propagation is ultimately terminated when two free radicals meet and either form a covalent bond joining the corresponding chains (combination) or having one chain transfer its unpaired electron to the other (disproportionation). Two other possibilities are as follows: (1) polymerization is terminated by stray free radicals that are present in the feed. Reactive oxygen species (ROS) are of particular concern, and care must be taken to minimize the presence of molecular oxygen, and (2) the propagating free radical extracts a hydrogen atom from another molecule, terminating the growing chain. However, a new free radical is formed on the “victim” molecule, initiates a new polymerization. This mechanism is called chain transfer and is sometimes used as a means to control molecular weight by addition of chain transfer agents. When the hydrogen atom is extracted from another polymer chain, a secondary branch may grow off the latter, creating a treelike or branched architecture.
Following initiation, which is often rate limiting, the propagation and termination steps are fast. Thus, individual chains are formed rather quickly and at different times within a polymerization run. This is in contrast to step-growth polymerization, in which all chains tend to grow at the same time.
Free radical polymerization, along with other addition polymerizations, possesses several advantages. It is relatively easy to carry out, and there exists a wide variety of vinylic monomers containing different side chains, which endow the polymer with desired properties. More than one monomer species can be included in a polymerization in order to fine-tune these properties or to confer two or more properties at once. Polymers containing more than one monomeric species are called copolymers. The structure of a copolymer depends on the rate that each monomer reacts with its own kind rather than with other types of monomers. When there are two types of monomers, chain propagation proceeds according to the following mechanism:
where k11, k12, k21, and k22 are the propagation rate constants. The reactivity ratios r1 = k11/k12 and r2 = k22/k21 provide information regarding the preference of monomers to react with their own kind and, hence, the tendency of monomers to be arranged (1) in blocks or (2) in alternating sequences. If r1 and r2 are much larger than unity, the likelihood of forming blocks of similar monomers increases, while if both are less than unity, the monomers tend to be arranged alternately. When the product r1r2 is close to unity, then the monomers are randomly incorporated in the copolymer chains, so long as the supply of both monomers remains adequate. It should be noted that unless special precautions are made, the monomer feed ratio will drift as the polymerization progresses, and there will be changes in monomer content.
Like step-growth polymerization, free radical polymerization produces chains whose lengths present a rather broad distribution. The reason for this is that the termination step can occur at any time, independent of the extent of propagation. Other kinds of addition polymerization can minimize such variability in chain length by suppressing the termination step. In anionic and cationic polymerizations, the growing chains have charged reaction centers at their growing ends, so termination by encounter between two chains does not occur. In ring-opening polymerization, the terminal monomer in a chain reacts with a cyclic-free monomer, which opens as it joins the polymer. Normally, there is no basis for interchain termination reactions.
In the past two decades, novel free radical polymerization schemes, including atom transfer radical polymerization (ATRP) and reversible addition-fragmentation chain transfer (RAFT) polymerization, have received much attention. In these schemes, most of the growing chain ends are “masked” by added reagents, slowing both the propagation and termination steps. Since termination is bimolecular, the likelihood of two unmasked chains finding each other and recombining or disproportionating is severely reduced. At the cost of slower polymerization, much more uniform polymer chain lengths are obtained.
Anionic, cationic, ring-opening, ATRP, and RAFT polymerizations are often called living, because the chain ends remain active even when the monomer supply is exhausted. It is therefore possible to produces block polymers, in which chains consisting of one kind of monomer serve as macroinitiators for another kind of chain. While typical applications of block polymers lie in the adhesive area, particular systems have also been shown to self-assemble into micellar and vesicular (polymersome) systems (see also Chapter 5, Section 5.5.3). As will be discussed in Section 14.2.5, properly constructed block polymers can also form physically cross-linked hydrogels.
14.2.3 STRUCTURAL CHARACTERISTICS OF LINEAR POLYMERS
We have already emphasized that the molecular weight of synthetic polymers is subject to random variation. Further structural variation can be introduced by copolymerization. Besides molecular weight and composition, another characteristic of a polymer is its tacticity. During an addition polymerization, the reactive center may be chiral with respect to the side chain, and sequential pairs of side chains may take on trans or cis configurations. Usually, this sequence of chiral additions is random, and the polymer is atactic. Chains in which all the side groups are added on the same side are called “isotactic,” whereas if the side groups alternate consistently, then the chain is considered syndiotactic. The latter two situations may require special polymerization conditions, e.g., catalysts, but there is a potential benefit insofar as the resulting polymers can form crystalline domains, or crystallites, by aligning with neighboring chains. Such crystalline domains form physical crosslinks, which may be stable even in the presence of solvent. For example, properly processed polyvinyl alcohol (PVA) can form stable hydrogels that absorb a substantial amount of water, even though there is no chemical cross-linking. Atactic polymers cannot crystallize. Similarly, copolymers must either consist of long homomonomeric blocks (blocks containing the same monomer) or long alternating sequences, in order to crystallize.
14.2.4 CHEMICALLY CROSS-LINKED NETWORKS
In Section 14.2.2.1, it was shown how step-growth polymers could be cross-linked by including multifunctional monomers. Irradiation followed by radical recombination was also mentioned as a general method for forming cross-links between polymer chains. In vinyl polymerization, the most common method of cross-linking is to include a small amount of a divinyl monomer into the polymerization reaction. As illustrated in Figure 14.6, this monomer can be incorporated into two independent growing chains. If several cross-linkers are incorporated into each chain, then the chains will have multiple connections to each other, forming a macroscopic network. Cross-linkers are incorporated randomly so that the distance between cross-links is random, the same as was pointed out for hydrogels formed by step-growth polymerization. Because of this randomness, important properties such as mesh size (Figure 14.2), which determines in part the diffusivity of molecules through the hydrogel, can only be characterized as averages.
A nonobvious characteristic of many hydrogels is their nonuniformity, which goes beyond the randomness seen in chemical structure distances between cross-links. Usually, hydrogels are synthesized in the presence of a solvent, such as water, in order to prevent overheating, which results from the exothermic nature of polymerization reactions. As monomers join to form polymers, they exhibit a tendency to phase separate from the solvent, forming small domains that are rich in polymer, alternating with regions that are rich in solvent. These variations in concentration are frozen in upon cross-linking. This phenomenon called “microsyneresis” may, if not controlled, produce a cloudy appearance. It can also lead to substantial weakening of the hydrogel structure and deviation in properties that are predicted assuming uniformity.
In recent years, there have been efforts to form “regular” hydrogels with better defined distances between cross-links and fewer problems with microsyneresis. One popular technique favored by those interested in preparing hydrogels for embedding of cells, e.g., for tissue engineering, is to use polyethylene glycol (PEG) chains that are terminated on both ends by vinyl (usually acrylate) groups. These macromers, which are reasonably monodisperse (nearly uniform in length), are “zipped” together by free radical polymerization of the end groups. Because the PEG chains are of nearly uniform length, are already in a polymerized state before cross-linking, and are hydrophilic, microsyneresis is reduced. Similar approaches involving modification of biopolymers such as dextrans with acrylate groups, followed by “zippering,” have been tried. Copolymers with a small fraction of side chains that can be cross-linked in response to light (photocross-linkers) can also be used to form uniform hydrogels, although the distance between cross-links in this case is more randomized. Most recently, nearly uniform length PEGs have been end-functionalized with groups that can condense with tetrafunctional RA-f (f = 4) cross-linkers, to form the so-called diamond hydrogels. Such hydrogels have been claimed to have “perfect” structure insofar as they are essentially free of inhomogeneities, and it has been shown that they have mechanical strengths greatly exceeding those of conventionally cross-linked hydrogels.
Since cross-links provide cohesiveness to the hydrogel, it follows that by incorporating biodegradable cross-linkers, the hydrogel can be programmed to lose its strength over time. This may be important for injectable and implantable systems, since it obviates the need to retrieve the hydrogel from the body after its purpose (e.g., for drug delivery or cell engraftment) has been served.
14.2.5 PHYSICALLY CROSS-LINKED NETWORKS
Physically cross-linked hydrogels can be formed using block polymers. Of particular interest are systems that are fluid at room temperature but self-assemble into 3D network structures at body temperature (Figure 14.7
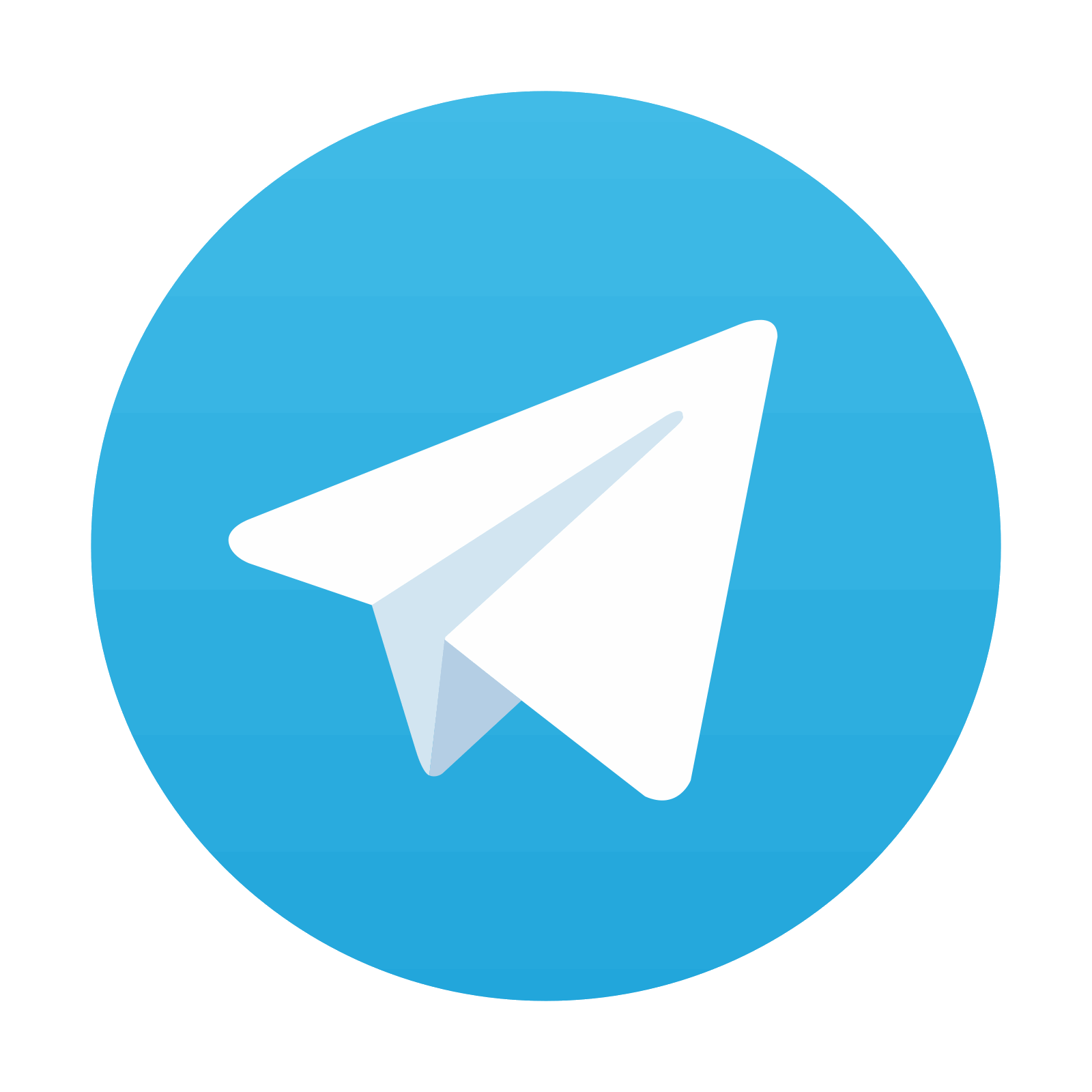
Stay updated, free articles. Join our Telegram channel
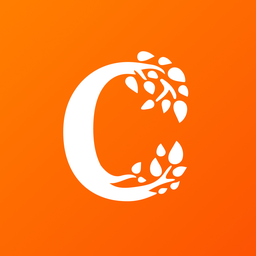
Full access? Get Clinical Tree
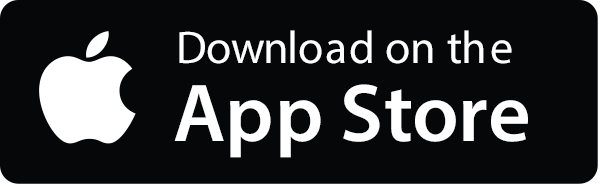
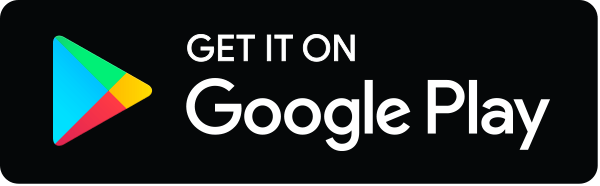