Glutamine1
Thomas R. Ziegler
1Abbreviations: ASPEN, American Society for Parenteral and Enteral Nutrition; ATP, adenosine triphosphate; BMT, bone marrow transplantation; EN, enteral nutrition; ESPEN, European Society for Enteral and Parenteral Nutrition; GH, growth hormone; GI, gastrointestinal; Glu, glutamate; GSH, glutathione; HE, hepatic encephalopathy; ICU, intensive care unit; ORS, oral rehydration solution; PN, parenteral nutrition; RCT, randomized controlled trial; SBS, short bowel syndrome; SCCM, Society of Critical Care Medicine; TCA, tricarboxylic acid
The amino acid glutamine, classically categorized as a nonessential amino acid, has become one of the most intensively studied nutrients in nutrition support research (1, 2, 3, 4, 5, 6, 7, 8, 9). Numerous studies in animal models of catabolic stress or intestinal mucosal injury support beneficial effects of parenteral or enteral glutamine supplementation (10, 11, 12). In addition, most, but not all, of the clinical outcome studies to date indicate that enteral and parenteral feedings supplemented with L-glutamine or glutamine dipeptides exert beneficial metabolic or clinical effects in various clinical conditions (3, 4, 5, 6, 7, 8, 9).
Glutamine is the most abundant amino acid in human blood and skeletal muscle as well as in the body’s total free amino acid pool (1, 2, 11, 13). Glutamine exhibits dynamic interorgan metabolism and is physiologically important in several central metabolic processes, including use as a preferential fuel (energy source) for intestinal mucosal and immune cells (10, 13, 14, 15, 16).
Several aspects of glutamine metabolism have direct relevance to nutrition support in clinical medicine, including strong evidence that glutamine becomes conditionally essential during certain catabolic states, when glutamine requirements in certain tissues exceed endogenous glutamine production and delivery to glutamine-using tissues (1, 6, 7, 8, 9, 11, 13, 14, 15, 16, 17, 18, 19, 20, 21). During illness, skeletal muscle exports large amounts of glutamine into the blood (>35% of all amino acid nitrogen) (17, 18, 19, 20). Concomitantly, glutamineusing tissues (e.g., the gut, kidney, and immune cells) markedly increase glutamine uptake and metabolism (1, 9, 11, 13, 14, 15, 16). When tissue glutamine use exceeds endogenous production, muscle glutamine levels fall, followed by a decrease in plasma levels, typically as a function of illness severity (1, 2, 20).
Provision of conventional parenteral nutrition (PN) or enteral nutrition (EN) by tube feedings or oral supplements does not adequately meet glutamine requirements in some patients during severe illness (see the later chapters on EN and PN for information on methods of specialized EN and PN support). However, exogenous glutamine, particularly when given intravenously, markedly affects protein anabolism in surgical and other types of catabolic patients (1, 2, 3, 4). In addition, in clinical randomized controlled trials (RCTs), primarily those comparing administration of glutamine-supplemented PN and EN with glutamine-free PN and low-glutamine EN, glutaminesupplemented PN has shown the greatest potential benefit in a wide range of catabolic clinical conditions (5, 6, 7, 8, 9).
BIOCHEMISTRY
As a classic nonessential amino acid, glutamine (Fig. 34.1) is synthesized endogenously in the cell cytoplasm from other amino acids, predominately branched-chain amino acids and glutamate (Glu) (16). Glutamine has two amine moieties, an α-amino group and a terminal amide group (1). Glutamine synthesis via Glu involves incorporation of ammonium ion, catalyzed by glutamine synthetase and driven by the hydrolysis of one adenosine triphosphate (ATP). Glutamine synthetase is particularly active in perivenous
hepatocytes, where it plays a major role in whole body glutamine production. The enzyme glutaminase is abundant in enterocytes (particularly in jejunum), in brain, kidney, and other tissues. In the cytoplasm of periportal hepatocytes, glutaminase is activated in response to increased portal vein concentrations of glutamine derived from the intestinal lumen (11).
hepatocytes, where it plays a major role in whole body glutamine production. The enzyme glutaminase is abundant in enterocytes (particularly in jejunum), in brain, kidney, and other tissues. In the cytoplasm of periportal hepatocytes, glutaminase is activated in response to increased portal vein concentrations of glutamine derived from the intestinal lumen (11).
Glutaminase cleaves the terminal amide group and catalyzes hydrolysis of glutamine to Glu and ammonium ion (see Fig. 34.1). The liver converts ammonia to urea, whereas Glu may be transaminated to form α-ketoglutarate, alanine, and aspartate (11, 16). The α-ketoglutarate can enter the tricarboxylic acid (TCA) cycle to generate energy; thus, complete oxidation of a mole of the five-carbon glutamine yields 30 mol of ATP, comparable to the 36 mol of ATP formed from oxidation of the six-carbon sugar glucose (1). Metabolism of glutamine by enterocytes yields carbon dioxide, alanine, ornithine, proline, and citrulline; glutamine also predominately serves as a nitrogen donor for citrulline synthesis within enterocytes (22, 23) (see later). Citrulline, in turn, participates in the synthesis of arginine by the kidney (22). Glutaminase activity is up-regulated to support gluconeogenesis during starvation (24), in which glutamine is one of the major gluconeogenic substrates (1, 18). During chronic or acute metabolic acidosis, the ammonium ion generated in kidney through glutamine hydrolysis by renal glutaminase (see Fig. 34.1) is excreted and thus serves to mitigate the acidosis (11, 16, 25).
DIETARY SOURCES
Glutamine is present in animal- and plant-derived proteins. Amino acid composition studies in foods have primarily used an acid hydrolysis method. A drawback is that hydrolysis of glutamine to Glu occurs before analysis. Thus, the specific glutamine content in most food protein and amino acid databases is not available, whereas the reported “Glu” content reflects the total content of glutamine plus Glu (26).
Amino acid composition of selected proteins using a gene sequencing method to calculate the percentage of specific amino acids in proteins revealed that glutamine comprised 8.9% of the total amino acids in cow’s milk β-casein, 3.8% in chicken egg ovalbumin, and 2.9% in skeletal muscle actin (composite preparation of human and several animal muscles) (1). Subsequent biochemical studies of protein-bound glutamine content in skeletal muscle from several species showed that glutamine comprised 4.8% and 4.1% of the amino acids in cow and pig muscle, respectively (27), whereas the glutamine content of whole proteins (soy, whey, casein) present in several commercial tube feeding formulations ranged from 5.18% to 7.89% of total protein content (28). Using gene sequencing methods, Lenders et al (26) analyzed the glutamine content of proteins in foods reported in the Nurse’s Health Study and found that the glutamine content of proteins in beef and skim milk was 1.2% and 2.8%, respectively, whereas that in white rice, soy (tofu), and hen’s egg equaled 1.2%, 6.0%, and 5.6 %, respectively. The total amounts of glutamine consumed in mixed meals or from nonsupplemented tube feeding formations (<8% of total protein) are much lower than the doses administered in clinical trials of glutamine supplementation in hospital settings, in which 20% to 40% of total administered protein and amino acids in PN or EN has been provided as glutamine (2, 3, 4, 5, 6, 7, 8, 9).
DIGESTION, ABSORPTION, AND TRANSPORT
Assimilation of dietary glutamine from the gastrointestinal (GI) tract occurs by enzymatic protein digestion and absorption, which in the healthy state are extremely efficient (28). Free L-glutamine is transported across enterocytes and other mammalian cell types by either sodium (Na+)-dependent or Na+-independent transport proteins (28, 29, 30, 31). Glutamine present in dipeptides and tripeptides after protein digestion is transported across the enterocyte apical membranes by the hydrogen (H+)-dependent transporter PepT1 (32). Na+-dependent transport uses the potential energy stored in Na+/potassium-ATPase to transport glutamine across transmembrane electrochemical gradients (28). The Na+-dependent glutamine transporter genes isolated thus far are System N subtypes (SN1, SNAT3, and SNAT5), System A (ATA1, ATA2), System ASC/B (0) (ASCT2 or ATB [0]), System B ([0, +] or ATB [0, +]) and System y+ L (y+LAT1, y+LAT2) (29, 30, 31). The SN1 transporter mediates the influx of two Na+ and one amino acid substrate per transport cycle coupled to the efflux of one H+ (29). Na+-independent glutamine transporter genes encoding for System L (LAT1, LAT2) and System b (0, +) [b (0, +) AT] have also been isolated (30). Most of these transporters also mediate transmembrane movement of other amino acids in addition to glutamine.
The splanchnic circulation is the primary source of glutamine for intestinal uptake (17), although acidosis
increases renal uptake significantly (33). Approximately 60% to 85% of the glutamine that is absorbed from the GI lumen of healthy humans is captured and presumably metabolized by the splanchnic bed (34, 35). Plasma glutamine concentrations rise in a dose-dependent fashion after oral glutamine loads (34, 35). In addition, enterally administered glutamine is metabolized by splanchnic tissues to amino acid end products. In one study, healthy adults given a single oral bolus dose of L-glutamine (0, 0.1, and 0.3 g/kg) showed a dose-related increase in plasma levels of alanine, citrulline, and arginine (converted from citrulline in kidney) (34). Tracer studies in healthy adults showed intestinal consumption of glutamine at a rate that depended on glutamine supply (36). Approximately 13% of glutamine taken up by the intestines was converted to citrulline, and glutamine was the only important precursor for intestinal citrulline release (36). In stable isotope studies, whole body glutamine use was reduced approximately 20% in patients with extensive small intestinal resection (37).
increases renal uptake significantly (33). Approximately 60% to 85% of the glutamine that is absorbed from the GI lumen of healthy humans is captured and presumably metabolized by the splanchnic bed (34, 35). Plasma glutamine concentrations rise in a dose-dependent fashion after oral glutamine loads (34, 35). In addition, enterally administered glutamine is metabolized by splanchnic tissues to amino acid end products. In one study, healthy adults given a single oral bolus dose of L-glutamine (0, 0.1, and 0.3 g/kg) showed a dose-related increase in plasma levels of alanine, citrulline, and arginine (converted from citrulline in kidney) (34). Tracer studies in healthy adults showed intestinal consumption of glutamine at a rate that depended on glutamine supply (36). Approximately 13% of glutamine taken up by the intestines was converted to citrulline, and glutamine was the only important precursor for intestinal citrulline release (36). In stable isotope studies, whole body glutamine use was reduced approximately 20% in patients with extensive small intestinal resection (37).
FUNCTIONS IN WHOLE BODY, ORGAN, AND CELLULAR METABOLISM
Important metabolic functions of glutamine are outlined in Table 34.1. In addition to its use as a precursor of TCA cycle intermediates for ATP generation as outlined earlier, glutamine is a key constituent of body proteins, comprising approximately 5% to 6% of bound amino acids. Glutamine plays a central role in amino acid transamination through α-ketoglutarate and Glu, in hepatic ureagenesis, and in ammonia detoxification (21, 38). Glutamine and Glu are the major nitrogen donors in the body. Glutamine is a major carrier of nitrogen in normal protein metabolism, and it accounts for approximately one third of the interorgan nitrogen that is shuttled in blood.
As a nitrogen donor for purine and pyrimidine synthesis, glutamine is critical for nucleotide, DNA, and RNA biosynthesis (1, 9, 21, 37). Glutamine has been well documented to be a primary and preferred energy substrate for rapidly dividing cell types, in particular enterocytes, colonocytes, and immune cells, especially lymphocytes and monocytes (1, 11, 14, 15, 21, 38, 39). The net proliferative effects of glutamine in these cell types are likely to result from the role of glutamine as a major fuel source, in purine and pyrimidine synthesis, and in stimulation of cell signaling pathways involved in cell proliferation and inhibition of apoptosis (1, 21, 40, 41). In addition, in vitro studies have shown that glutamine increases the size of hepatocytes and other cell types through osmosignaling mechanisms, thus resulting in antiprotein catabolic effects (21, 42).
TABLE 34.1 MAJOR METABOLIC FUNCTIONS OF GLUTAMINE | |||||||
---|---|---|---|---|---|---|---|
|
As noted earlier, glutamine and alanine, derived predominately from skeletal muscle, are the body’s major amino acid gluconeogenic precursors (1, 21). Various studies suggested that glutamine contributes to glucose homeostasis in other ways, including as an insulin secretagogue and by enhancing insulin sensitivity and thereby increasing glucose uptake (21, 43, 44, 45). The nitrogen in the side chain of glutamine also contributes to the biosynthesis of a broad range of metabolic compounds (1, 11, 21); and Glu, formed through hydrolysis of glutamine by glutaminase, itself serves critical metabolic functions, including as a neurotransmitter, a precursor to glutamine, proline, and arginine, in amino acid transamination reactions, and as a component of the major tripeptide antioxidant glutathione (GSH), among others.
GLUTAMINE DEPLETION DURING CATABOLIC STATES
Glutamine exhibits dynamic interorgan metabolism, particularly between skeletal muscle and the splanchnic bed and kidney—the major sites of glutamine uptake during illness. The concentration of glutamine in plasma and muscle pools is labile during catabolic states, and a marked decrease in glutamine concentration occurs first in muscle and later in plasma (1, 19, 20). In humans after uncomplicated moderate intra-abdominal surgical procedures, the free intracellular glutamine concentration in skeletal muscle falls within a few days to 25% to 60% of preoperative values (6); in patients with severe pancreatitis or sepsis, muscle glutamine levels fall even more dramatically, sometimes to less than 20% of normal values (9, 20). Under these conditions, skeletal muscle exports large amounts of glutamine into blood, up to more than 35% of all amino acid nitrogen in some clinical settings (1, 6, 7, 9, 17, 18, 19, 20). Concomitantly, as shown in animal models of catabolic stress, glutamine-using tissues including the gut, kidney, and immune cells markedly increase their uptake and metabolism of glutamine (1, 6, 7, 9, 11, 14, 15, 16, 46). When tissue glutamine use exceeds endogenous production, the drop in muscle glutamine is followed by a decrease in plasma glutamine, typically as a function of illness severity (1, 2, 7, 20). Decreased glutamine in muscle and plasma is associated with worse clinical outcomes; however, whether this is a function of the severity of depletion or whether glutamine depletion is simply a biomarker for severe illness is unclear (5, 6, 7, 47). Conventional PN formulations, and standard low-glutamine EN by tube feedings or oral supplements, do not adequately meet the glutamine
requirements of some patients during catabolic illness because glutamine is absent in standard PN and present in low quantities in most EN formulations (1, 7, 9). Elegant interorgan studies by Souba et al (10, 11, 16) showed that following operative trauma, the efflux of glutamine from the lung and, especially, from muscle was accelerated to provide glutamine to wounds, the gut, immune cells, kidneys (for renal ammoniagenesis), and liver. These effects were mediated by the rise that occurs in glucocorticosteroids in blood. In sepsis, a more dramatic release of glutamine from muscle and, to a lesser extent, from lung was noted. Immune system cells and liver appeared to be the major consumers of glutamine, whereas glutamine uptake in the gut and kidney was diminished (10, 11, 16). Studies in humans confirmed that high doses of glucocorticoids increase glutamine extraction by the splanchnic bed, presumably secondary to a regional increase in glutamine requirements (16, 17).
requirements of some patients during catabolic illness because glutamine is absent in standard PN and present in low quantities in most EN formulations (1, 7, 9). Elegant interorgan studies by Souba et al (10, 11, 16) showed that following operative trauma, the efflux of glutamine from the lung and, especially, from muscle was accelerated to provide glutamine to wounds, the gut, immune cells, kidneys (for renal ammoniagenesis), and liver. These effects were mediated by the rise that occurs in glucocorticosteroids in blood. In sepsis, a more dramatic release of glutamine from muscle and, to a lesser extent, from lung was noted. Immune system cells and liver appeared to be the major consumers of glutamine, whereas glutamine uptake in the gut and kidney was diminished (10, 11, 16). Studies in humans confirmed that high doses of glucocorticoids increase glutamine extraction by the splanchnic bed, presumably secondary to a regional increase in glutamine requirements (16, 17).
CLINICAL AND METABOLIC IMPACT OF GLUTAMINE SUPPLEMENTATION IN DISEASE STATES
Cytoprotective Effects
Numerous studies have demonstrated that exogenous glutamine supplementation exerts effects that can be considered cytoprotective and include protein anabolic effects and improved nitrogen balance after major surgery (2, 5, 7, 8, 13, 48, 49, 50, 51, 52). Glutamine has been shown to stimulate cell proliferation and inhibited apoptosis in intestinal epithelial cells (12, 40, 41); enhance endogenous immune function (6, 7, 9, 15); up-regulate the synthesis of GSH, inhibited oxidative stress, and improved redox control (53, 54, 55, 56, 57, 58, 59); enhance generation of cytoprotective heat shock proteins (HSPs) (60, 61, 62, 63); preserve gut mucosal tight junction protein structure and function (12, 64, 65, 66, 67); up-regulate intestinal secretory immunoglobulin A (67); and decrease proinflammatory cytokine responses (63, 68), among others (69, 70). Cytoprotective effects and potential mechanisms with glutamine supplementation observed in cell, animal, and human models of catabolic stress are listed in Table 34.2. Detailed discussions of glutamine cytoprotectivity can be found in earlier publications (2, 7, 8, 9, 12, 13, 14, 40, 41, 48, 49, 50, 51, 52, 53, 54, 55, 56, 57, 58, 59, 60, 61, 62, 63, 64, 65, 66, 67, 68, 69, 70).
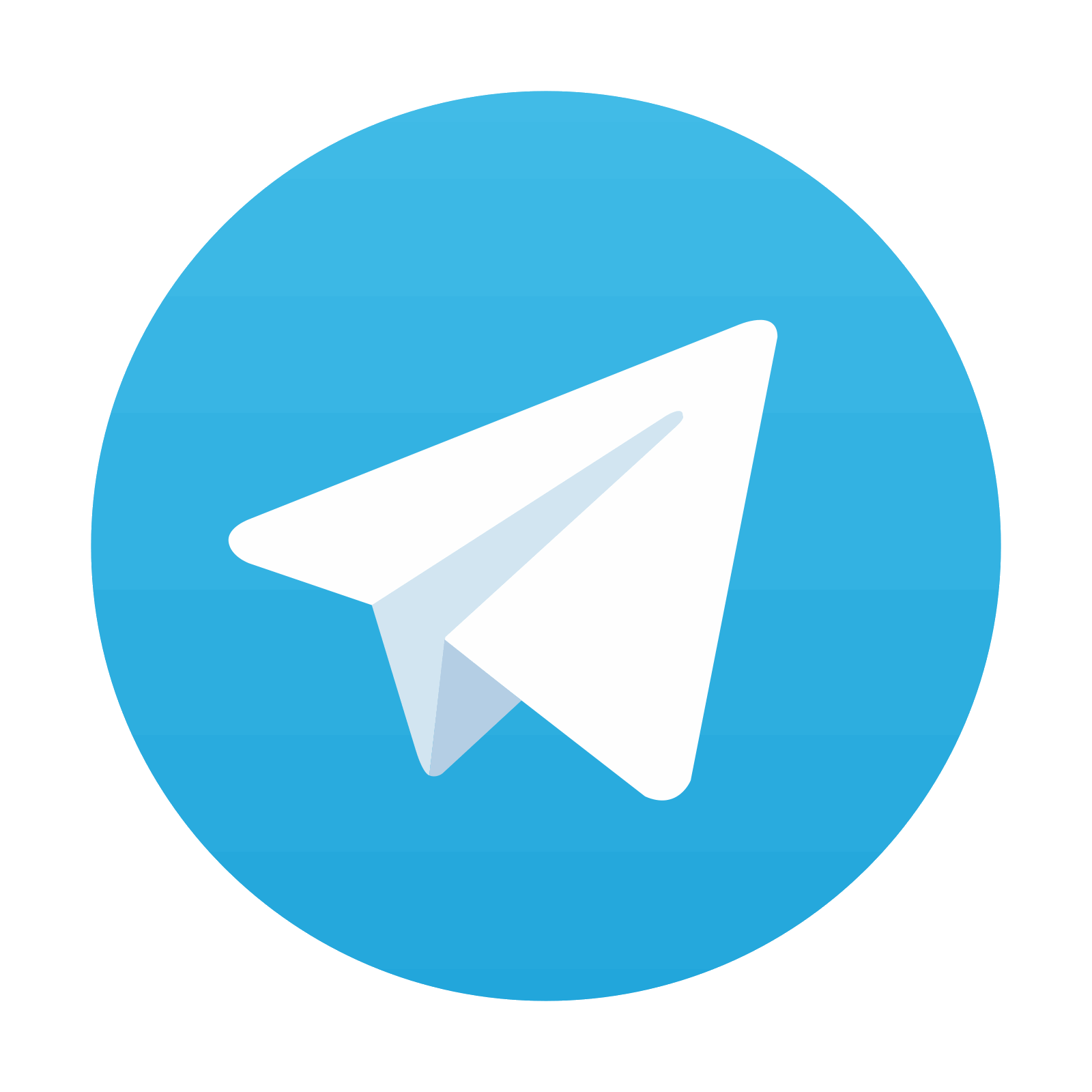
Stay updated, free articles. Join our Telegram channel
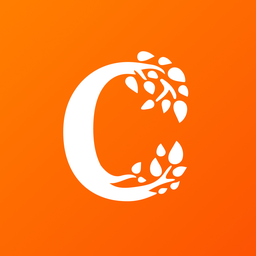
Full access? Get Clinical Tree
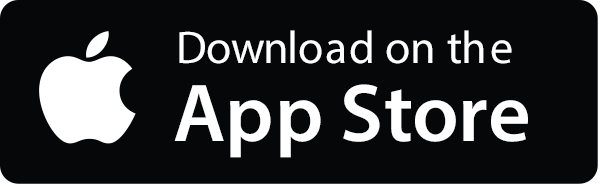
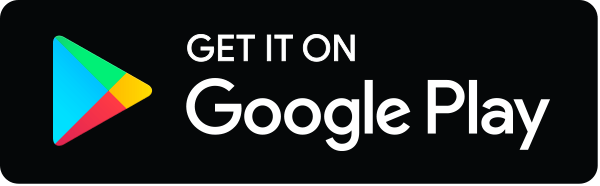