20
Gluconeogenesis & the Control of Blood Glucose
David A. Bender, PhD & Peter A. Mayes, PhD, DSc
OBJECTIVES
After studying this chapter, you should be able to:
Explain the importance of gluconeogenesis in glucose homeostasis.
Describe the pathway of gluconeogenesis, how irreversible enzymes of glycolysis are bypassed, and how glycolysis and gluconeogenesis are regulated reciprocally.
Explain how plasma glucose concentration is maintained within narrow limits in the fed and fasting states.
BIOMEDICAL IMPORTANCE
Gluconeogenesis is the process of synthesizing glucose or glycogen from noncarbohydrate precursors. The major substrates are the glucogenic amino acids (Chapter 29), lactate, glycerol, and propionate. Liver and kidney are the major gluconeogenic tissues; the kidney may contribute up to 40% of total glucose synthesis in the fasting state and more in starvation. The key gluconeogenic enzymes are expressed in the small intestine, but it is unclear whether or not there is significant glucose production by the intestine in the fasting state.
A supply of glucose is necessary especially for the nervous system and erythrocytes. After an overnight fast, glycogenolysis (Chapter 19) and gluconeogenesis make approximately equal contributions to blood glucose; as glycogen reserves are depleted, so gluconeogenesis becomes progressively more important.
Failure of gluconeogenesis is usually fatal. Hypoglycemia causes brain dysfunction, which can lead to coma and death. Glucose is also important in maintaining the level of intermediates of the citric acid cycle even when fatty acids are the main source of acetyl-CoA in the tissues. In addition, gluconeogenesis clears lactate produced by muscle and erythrocytes, and glycerol produced by adipose tissue. In ruminants, propionate is a product of rumen metabolism of carbohydrates, and is a major substrate for gluconeogenesis.
Excessive gluconeogenesis occurs in critically ill patients in response to injury and infection, contributing to hyperglycemia which is associated with a poor outcome. Hyperglycemia leads to changes in osmolality of body fluids, impaired blood flow, intracellular acidosis and increased superoxide radical production (Chapter 45), resulting in deranged endothelial and immune system function and impaired blood coagulation. Excessive gluconeogenesis is also a contributory factor to hyperglycemia in type 2 diabetes because of impaired sensitivity of gluconeogenesis to downregulation in response to insulin.
GLUCONEOGENESIS INVOLVES GLYCOLYSIS, THE CITRIC ACID CYCLE, PLUS SOME SPECIAL REACTIONS
Thermodynamic Barriers Prevent a Simple Reversal of Glycolysis
Three nonequilibrium reactions in glycolysis (Chapter 18), catalyzed by hexokinase, phosphofructokinase and pyruvate kinase, prevent simple reversal of glycolysis for glucose synthesis (Figure 20–1). They are circumvented as follows.
FIGURE 20–1 Major pathways and regulation of gluconeogenesis and glycolysis in the liver. Entry points of glucogenic amino acids after transamination are indicated by arrows extended from circles (see also Figure 17–4). The key gluconeogenic enzymes are enclosed in double-bordered boxes. The ATP required for gluconeogenesis is supplied by the oxidation of fatty acids. Propionate is of quantitative importance only in ruminants. Arrows with wavy shafts signify allosteric effects; dash-shafted arrows, covalent modification by reversible phosphorylation. High concentrations of alanine act as a “gluconeogenic signal” by inhibiting glycolysis at the pyruvate kinase step.
Pyruvate & Phosphoenolpyruvate
Reversal of the reaction catalyzed by pyruvate kinase in glycolysis involves two endothermic reactions. Mitochondrial pyruvate carboxylase catalyzes the carboxylation of pyruvate to oxaloacetate, an ATP-requiring reaction in which the vitamin biotin is the coenzyme. Biotin binds CO2 from bicarbonate as carboxybiotin prior to the addition of the CO2 to pyruvate (Figure 44–17). The resultant oxaloacetate is reduced to malate, exported from the mitochondrion into the cytosol and there oxidized back to oxaloacetate. A second enzyme, phosphoenolpyruvate carboxykinase, catalyzes the decarboxylation and phosphorylation of oxaloacetate to phosphoenolpyruvate using GTP as the phosphate donor. In liver and kidney, the reaction of succinate thiokinase in the citric acid cycle (Chapter 17) produces GTP (rather than ATP as in other tissues), and this GTP is used for the reaction of phosphoenolpyruvate carboxykinase, thus providing a link between citric acid cycle activity and gluconeogenesis, to prevent excessive removal of oxaloacetate for gluconeogenesis, which would impair citric acid cycle activity.
Fructose 1,6-Bisphosphate & Fructose 6-Phosphate
The conversion of fructose 1,6-bisphosphate to fructose 6-phosphate, for the reversal of glycolysis, is catalyzed by fructose 1,6-bisphosphatase. Its presence determines whether a tissue is capable of synthesizing glucose (or glycogen) not only from pyruvate, but also from triose phosphates. It is present in liver, kidney, and skeletal muscle, but is probably absent from heart and smooth muscle.
Glucose 6-Phosphate & Glucose
The conversion of glucose 6-phosphate to glucose is catalyzed by glucose 6-phosphatase. It is present in liver and kidney, but absent from muscle and adipose tissue, which, therefore, cannot export glucose into the bloodstream.
Glucose 1-Phosphate & Glycogen
The breakdown of glycogen to glucose 1-phosphate is catalyzed by phosphorylase. Glycogen synthesis involves a different pathway via uridine diphosphate glucose and glycogen synthase (Figure 19–1).
The relationships between gluconeogenesis and the glycolytic pathway are shown in Figure 20–1. After transamination or deamination, glucogenic amino acids yield either pyruvate or intermediates of the citric acid cycle. Therefore, the reactions described above can account for the conversion of both lactate and glucogenic amino acids to glucose or glycogen.
Propionate is a major precursor of glucose in ruminants; it enters gluconeogenesis via the citric acid cycle. After esterification with CoA, propionyl-CoA is carboxylated to D-methylmalonyl-CoA, catalyzed by propionyl-CoA carboxylase, a biotin-dependent enzyme (Figure 20–2). Methylmalonyl-CoA racemase catalyzes the conversion of D-methylmalonyl-CoA to L-methylmalonyl-CoA, which then undergoes isomerization to succinyl-CoA catalyzed by methylmalonyl-CoA mutase. In nonruminants, including human beings, propionate arises from the β-oxidation of odd-chain fatty acids that occur in ruminant lipids (Chapter 22), as well as the oxidation of isoleucine and the side chain of cholesterol, and is a (relatively minor) substrate for gluconeogenesis. Methylmalonyl-CoA mutase is a vitamin B12-dependent enzyme, and in deficiency methylmalonic acid is excreted in the urine (methylmalonicaciduria).
FIGURE 20–2 Metabolism of propionate.
Glycerol is released from adipose tissue as a result of lipolysis of lipoprotein triacylglycerol in the fed state; it may be used for reesterification of free fatty acids to triacylglycerol in adipose tissue or liver, or may be a substrate for gluconeogenesis in the liver. In the fasting state, glycerol released from lipolysis of adipose tissue triacylglycerol is used solely as a substrate for gluconeogenesis in the liver and kidneys.
SINCE GLYCOLYSIS & GLUCONEOGENESIS SHARE THE SAME PATHWAY BUT IN OPPOSITE DIRECTIONS, THEY MUST BE REGULATED RECIPROCALLY
Changes in the availability of substrates are responsible for most changes in metabolism either directly or indirectly acting via changes in hormone secretion. Three mechanisms are responsible for regulating the activity of enzymes concerned in carbohydrate metabolism: (1) changes in the rate of enzyme synthesis, (2) covalent modification by reversible phosphorylation, and (3) allosteric effects.
Induction & Repression of Key Enzymes Requires Several Hours
The changes in enzyme activity in the liver that occur under various metabolic conditions are listed in Table 20-1. The enzymes involved catalyze nonequilibrium (physiologically irreversible) reactions. The effects are generally reinforced because the activity of the enzymes catalyzing the reactions in the opposite direction varies reciprocally (see Figure 20–1). The enzymes involved in the utilization of glucose (ie, those of glycolysis and lipogenesis) become more active when there is a superfluity of glucose, and under these conditions the enzymes of gluconeogenesis have low activity. Insulin, secreted in response to increased blood glucose, enhances the synthesis of the key enzymes in glycolysis. It also antagonizes the effect of the glucocorticoids and glucagon-stimulated cAMP, which induce synthesis of the key enzymes of gluconeogenesis.
TABLE 20–1 Regulatory and Adaptive Enzymes Associated with Carbohydrate Metabolism
Covalent Modification by Reversible Phosphorylation Is Rapid
Glucagon and epinephrine, hormones that are responsive to a decrease in blood glucose, inhibit glycolysis and stimulate gluconeogenesis in the liver by increasing the concentration of cAMP. This in turn activates cAMP-dependent protein kinase, leading to the phosphorylation and inactivation of pyruvate kinase. They also affect the concentration of fructose 2,6-bisphosphate and therefore glycolysis and gluconeogenesis, as described below.
Allosteric Modification Is Instantaneous
In gluconeogenesis, pyruvate carboxylase, which catalyzes the synthesis of oxaloacetate from pyruvate, requires acetyl-CoA as an allosteric activator. The addition of acetyl-CoA results in a change in the tertiary structure of the protein, lowering the Km
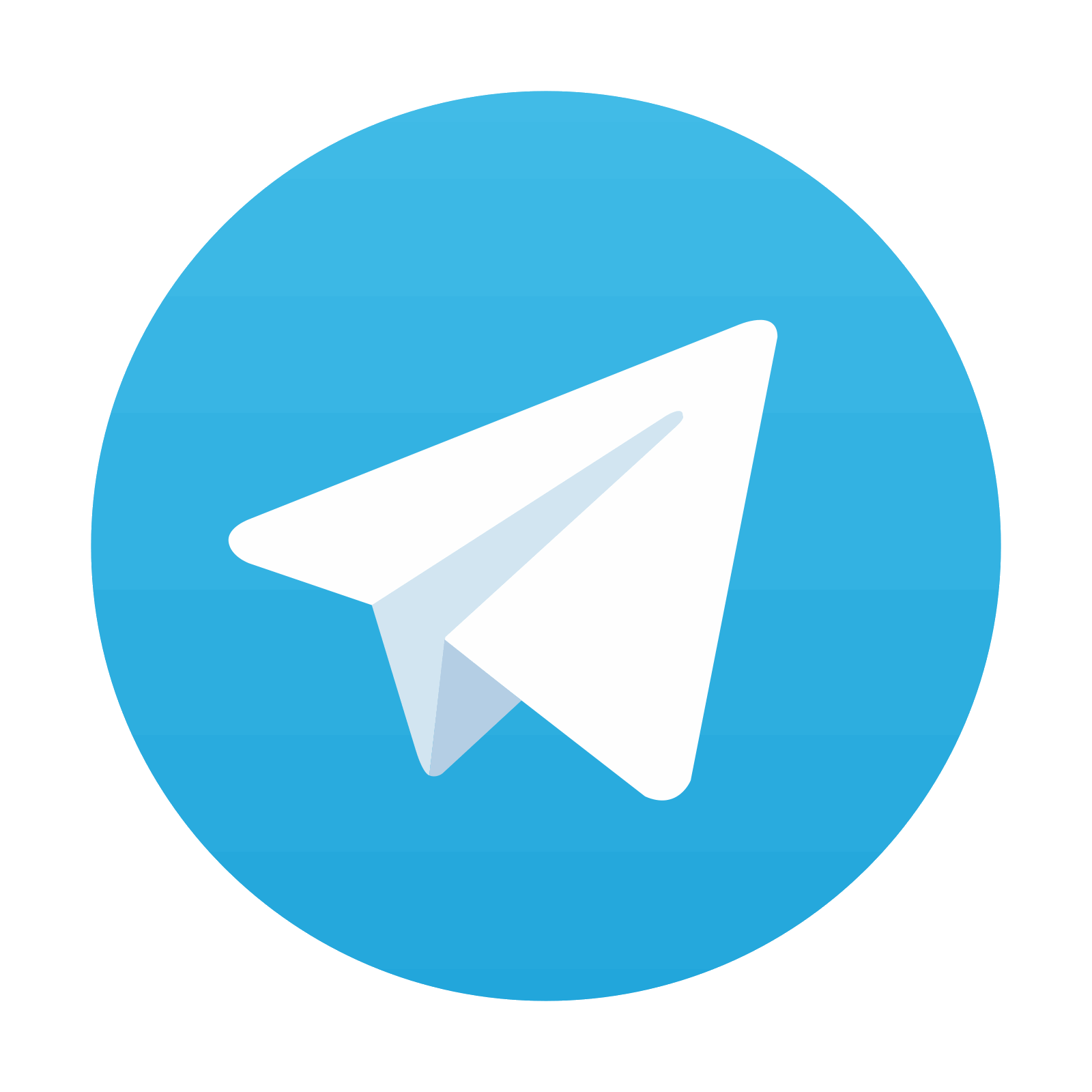
Stay updated, free articles. Join our Telegram channel
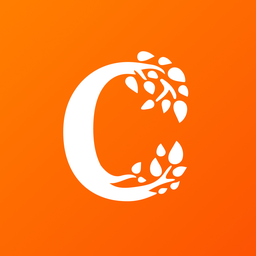
Full access? Get Clinical Tree
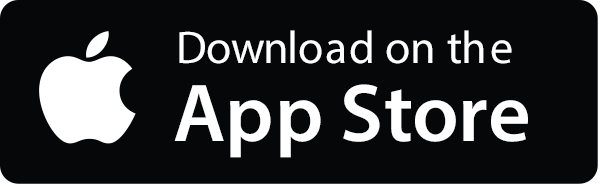
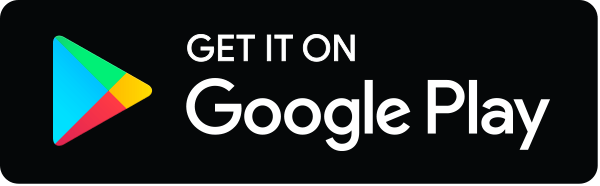