Genetics
Genetics is the study of genes. Genes are biological units that direct the synthesis of specific proteins. Genes are located inside chromosomes that contain the deoxyribonucleic acid (DNA) that transmits hereditary characteristics. Genes are passed from parent to offspring and determine identity and function at the most basic cellular level. Transmission of genetic information is a balance between ensuring that genes are passed error-free between the generations and allowing enough diversity for the adaptation and survival of the species. Sometimes mistakes (mutations) are made that advance the species—for example, mutations have resulted in several malaria-protective genes that offer evolutionary advantage, but in most cases mutations cause significant disability or death.
Genomics, the study of multiple genes and how they interact with the environment, offers insight into the study of complex diseases. Since the initiation of the Human Genome Project (HGP), specific genes for diseases such as cystic fibrosis, diabetes, Huntington disease, and some inheritable types of breast and colon cancer have been identified. It is anticipated that additional research will be helpful in identifying the most effective cancer treatment with the least potential for toxicity.
● The Human Genome Project
In 1987, the United States Congressional advisory committee recommended a multidisciplinary, scientific, and technological endeavor aimed at sequencing the human genome (all DNA contained in an organism). This recommendation resulted in the HGP that began in 1989 and offered insight into the link between genes and numerous diseases.
APPLICATIONS
According to the National Institute of Health, this 13-year project has resulted in the identification of over 1800 genes linked to diseases and the development of approximately 1000 genetic tests.
The project has further prompted extensive research into the use of gene therapy in which viruses are used to carry genes into the cells. For example, in two separate studies gene transfer therapy was found to be effective in halting retinal degeneration and improving visual acuity in young adults with Leber’s congenital amaurosis (optic neuropathy). Gene therapy is designed to replace missing or defective genes. As a result of the HGP, the HapNap project is now focusing on various diseases worldwide. As research continues, the focus will be on genomic scanning to prevent and/or diagnose early, perfecting gene therapy, and gaining more insight into drug discovery based on specific gene targets. Some predict that within 20 years, patients entering a medical facility will be subjected to a genomic scanning for pathogenic and “epistatic” genes. Epistasis refers to the tendency of some genes to interact and cross over with genes other than their allelic partners. Epistasis appears to affect certain diseases positively or negatively and has the potential to result in variable intensities of disease in different ethnicities and individuals.
One area of genomics that has progressed more slowly than anticipated is that involving drug discovery based on specific gene targets unveiled by the HGP. Progress in this area has been slow for a number of reasons. First, the complexity of the human physiologic system has proven to be a bit daunting, perhaps accounting for the fact that most of the highly effective drugs on the market today, such as aspirin, act on multiple gene systems rather than on single-gene disorders. The tendency of genes to experience epistatic interactions has also hindered the pace of drug production through gene targeting.
ETHICAL IMPLICATIONS
Results of the HGP will have enormous ethical implications for prenatal testing and selective abortion of defective embryos. Ethical implications are also involved in testing concerned adults who seek to know the likelihood of their developing a specific disease, such as Huntington disease, in the future. This is especially troubling if the identified disease is one for which there is no treatment or cure, or if testing involves children. For adults and children with disease-causing mutations, future childbearing choices, the ability to purchase health insurance or life insurance, and the ability to find future employment are important considerations. The HGP has dedicated funding and time to explore the ethical factors involved in gene mapping. In the fall of 2005, a study was initiated by the National Human Genome Research Institute among healthy adult volunteers to sequence 100 to 300 genes that have been associated with various disease phenotypes. The aim is to provide information to
individuals on potential genetic risk factors and to evaluate how this enormous amount of information is handled by the patients and their families.
individuals on potential genetic risk factors and to evaluate how this enormous amount of information is handled by the patients and their families.
● Physiologic Concepts
GENES
There are approximately 25,000 genes in the human genome, with each gene containing a few hundred to a few thousand base pairs of DNA. This is a much smaller number of genes than expected, given the complexity of the human genome. The DNA of a given gene includes coding portions, called exons, and noncoding portions. The coding portions of a gene carry the information needed to make a protein, often an enzyme. Enzymes and other proteins control the synthesis and function of each and every cell or tissue of the body. The function of the millions of molecules of noncoding DNA is unclear. Many genes grouped together make up the chromosomes.
CHROMOSOMES
Chromosomes are made up of molecules of DNA, complexed with proteins called histones. Chromosomes carry the genetic blueprint of an individual. All human somatic (body) cells contain 23 pairs of chromosomes, one pair from each parent, for a total of 46 chromosomes. Of the 23 pairs of chromosomes, 22 are the same in both sexes. The 23rd pair consists of the sex chromosome, X or Y. The female has two X chromosomes and the male has one X and one Y. Each human sex cell, an egg or a sperm, contains 23 unpaired chromosomes. Each chromosome is nearly identical (approximately 99.9%) across the human species in the genetic information it contains. The remaining variations are subtle but enough to make each one of us unique.
GENE ACTIVATION
Although each somatic cell contains the same 23 pairs of chromosomes, only certain genes are activated in any given cell; therefore, only certain proteins or enzymes are produced by that cell. Which genes are activated in which cell is determined during embryologic development and throughout life by circulating growth factors, hormones, and chemical cues produced by a given cell and its neighboring cells. In addition, methylation of certain regions of a gene (adding a CH3 group) can turn off, or silence, that gene. Demethylating the region can activate that gene and lead to the production of the protein for which it codes. In general, cells that have similar genes turned on and off perform similar functions and group together as tissues.
It has recently become clear that many genes can code for more than one protein, a finding that helps explain how so few genes can result in so many
different proteins. Recent research suggests that a process known as “alternative splicing” is responsible for this phenomenon. Alternative splicing refers to different combinations of exons on one gene that become active at different times, with each combination resulting in the production of one protein.
different proteins. Recent research suggests that a process known as “alternative splicing” is responsible for this phenomenon. Alternative splicing refers to different combinations of exons on one gene that become active at different times, with each combination resulting in the production of one protein.
DNA
Each chromosome is composed of hundreds of thousands of molecules of DNA. DNA is made up of phosphoric acid, a sugar molecule called deoxyribose, and one of four possible nitrogen bases: adenine, guanine, cytosine, or thymine. DNA molecules line up in the cell in the form of a double helix (Fig. 2-1) DNA, in which the phosphoric acid and the deoxyribose sugar form
the backbone of the helix. The base pairs from two molecules of DNA lie between the two strands of the helix, opposing each other. Adenine always bonds across the helix with thymine, and cytosine always bonds with guanine. The bonds are loose, so that the helixes can separate when cell division occurs or when protein synthesis is initiated.
the backbone of the helix. The base pairs from two molecules of DNA lie between the two strands of the helix, opposing each other. Adenine always bonds across the helix with thymine, and cytosine always bonds with guanine. The bonds are loose, so that the helixes can separate when cell division occurs or when protein synthesis is initiated.
● Cellular Reproduction
All cells reproduce during embryonic development, which allows for the growth of the embryo and differentiation (specialization) of the cells making up tissues and organs. After birth and throughout adulthood, many cells continue to reproduce. Cells that reproduce throughout a lifetime include cells of the bone marrow, skin, and digestive tract. Liver and kidney cells reproduce when replacement of lost or destroyed cells is required. Special cells, called stem cells, are capable of reproducing indefinitely. Other cells, including nerve, skeletal muscle, and cardiac muscle cells, do not reproduce significantly after the first few months following birth. Damage to these tissues generally cannot be repaired by the growth of new cells (although nearby stem cells may differentiate into replacement cells). To reproduce, a cell has to replicate its genetic material and then split in two. Replication and division of a cell occur during the cell cycle.
REPLICATION
To replicate, the DNA double helix uncoils and each strand of DNA serves as a template for a new strand. In the formation of a new strand of DNA, each adenine will bind only with a thymine and each cytosine will pair only with guanine. Therefore, only one strand, acting as a mirror-image template for the other, is needed to replicate the entire double helix.
Replication of the chromosome pairs and the DNA occurs in the nucleus of the cell. Various enzymes participate in DNA replication, which results in each chromosome being exactly copied or duplicated. The duplication is checked and double-checked by several proofreading enzymes to ensure that no mistakes are made. If a mistake is identified, proofreading or other enzymes remove the error and correct it, or the cell may initiate its own death in a process called apoptosis. If a mistake is not repaired, and the cell does not undergo apoptosis, a mutation in the DNA will exist.
THE CELL CYCLE
The cell cycle (Fig. 2-2) refers to a sequence of stages that a cell goes through during its lifetime. During embryogenesis, all cells go through all stages, as
do adult cells that continue to reproduce. The rate at which a cell goes through its cell cycle depends on the given cell and the growth factors, hormones, and chemicals to which it is exposed. Cells that do not continue to reproduce after embryogenesis remain in a resting stage and do not cycle through the other stages. The cell cycle is divided into two parts: interphase and mitosis.
do adult cells that continue to reproduce. The rate at which a cell goes through its cell cycle depends on the given cell and the growth factors, hormones, and chemicals to which it is exposed. Cells that do not continue to reproduce after embryogenesis remain in a resting stage and do not cycle through the other stages. The cell cycle is divided into two parts: interphase and mitosis.
Interphase
When not actively dividing, a cell is said to be in interphase. There are three standard stages of interphase: G1, S, and G2. A fourth stage, G0, is a specialized resting stage. In these designations, the G stands for gap, referring to a time period that the cell uses to check and recheck the preceding steps.
G1 is the stage during which a cell prepares for DNA replication by synthesizing new proteins and activating cytoskeletal components. During this stage, the cell monitors its environment to determine if the time is right for DNA replication. This stage is considered a checkpoint for the cell because
if conditions are not right, the cell will not progress further through its cycle. A cell will be stimulated to progress through G1 when certain genes, including proto-oncogenes, are activated. S is the next stage, during which replication (copying) of the DNA occurs; DNA replication is described in Chapter 1. The third stage, G2, is the stage before cell division, during which the cell again undergoes protein synthesis, this time in preparation for division. This stage is also a checkpoint because if the DNA has not been copied correctly, the cell has a second opportunity to stop its progression through the cycle before mitosis occurs. If an error has occurred, either it is repaired, and the cell re-enters the cell cycle, or the cell is stimulated to undergo apoptosis, i.e., programmed cell death. Genes that are activated at this stage to stop the progression of the cell through its cycle are known as tumor suppressor genes.
if conditions are not right, the cell will not progress further through its cycle. A cell will be stimulated to progress through G1 when certain genes, including proto-oncogenes, are activated. S is the next stage, during which replication (copying) of the DNA occurs; DNA replication is described in Chapter 1. The third stage, G2, is the stage before cell division, during which the cell again undergoes protein synthesis, this time in preparation for division. This stage is also a checkpoint because if the DNA has not been copied correctly, the cell has a second opportunity to stop its progression through the cycle before mitosis occurs. If an error has occurred, either it is repaired, and the cell re-enters the cell cycle, or the cell is stimulated to undergo apoptosis, i.e., programmed cell death. Genes that are activated at this stage to stop the progression of the cell through its cycle are known as tumor suppressor genes.
G0 is a resting stage in which a cell in G1 that has not committed itself to DNA replication may pause. A cell may stay in Go indefinitely, but once a cell is stimulated to leave the Go stage, it will progress through the other stages, unless its progress is restricted at a subsequent checkpoint. The progression through interphase is a lengthy 10- to 22-hour process.
Mitosis
Mitosis (the M stage) is the stage of cell division (Fig. 2-3). Mitosis is a shorter event than interphase; it lasts approximately 1 hour. During mitosis, the cell that has duplicated during interphase splits into two daughter cells each of which contains the 23 pairs of chromosomes. Mitosis consists of the substages of prophase, metaphase, anaphase, and telophase.
Prophase
Prophase is the stage in which protein structures (centrioles) present in the cytoplasm of the cell begin to move toward opposite sides or poles of the cell. This stretches the nuclear membrane and causes it to break apart. The chromosomes are now in the cytoplasm rather than isolated in the nucleus.
Metaphase
Metaphase is the stage during which the chromosomes visibly become two sets of pairs lined up next to each other in the center of the cell. Microtubules extend from the centrioles to each chromosome pair.
Anaphase
Anaphase is the stage during which the microtubules begin to pull the chromosome pairs apart. One chromosome goes toward one centriole pole and the other goes toward the other centriole pole.
Telophase
Telophase is the stage during which the cell splits down the middle and a new nuclear membrane develops around each of the two new cells, including the 23 pairs of chromosomes (46 in total) present in each cell.
Control of the Cell Cycle
Cells that continually go through the cell cycle (i.e., cells of the gut and bone marrow) do so at an intrinsic rate that can be increased or decreased by internal and external cues. External cues that turn on the cell cycle may include neural or hormonal stimulation, or may come from cell products released in response to tissue injury and activation of the inflammatory and immune systems. Brakes on the cell cycle may also include neural and hormonal stimulation and proteins synthesized by cells in response to activation of certain
regulator genes, including the tumor suppressor genes. Uncontrollable cell growth and cancer (see Chapter 3) may occur with the destruction or inactivation of regulator genes or by excessive stimulation and activation of proto-oncogenes.
regulator genes, including the tumor suppressor genes. Uncontrollable cell growth and cancer (see Chapter 3) may occur with the destruction or inactivation of regulator genes or by excessive stimulation and activation of proto-oncogenes.
Another mechanism that serves to limit cell replication involves structures present on the chromosomes themselves, known as telomeres. A telomere is the end region of a chromosome that shortens with each replication. When the telomere shortens to a threshold length, after a certain number of cell cycles, it shuts off cellular replication. Shutting off cellular replication leads to replicative senescence, the characteristic that ensures normal somatic cells do not divide indefinitely. However, a few cells, including cancer cells and germ line cells, contain the enzyme telomerase; telomerase adds telomere sections back onto the chromosome. Telomerase stabilizes telomere length, which results in immortalizing these cells. Telomerase is discussed further in Chapter 3.
MEIOSIS
Meiosis is the process during which germ cells of the ovary (primary oocytes) or testicle (primary spermatocytes) give rise to mature eggs or sperm (Fig. 2-4). Meiosis involves DNA replication in the germ cell, followed by two cell divisions rather than one, which results in four daughter cells, each with 23 (unpaired) chromosomes. In males, all four daughter cells are viable and continue to differentiate into mature sperm. In females, only one viable daughter cell (egg) is formed; the other three cells become nonfunctional polar bodies. During fertilization, genetic information contained in the 23 chromosomes of the egg joins with genetic information contained in the 23 chromosomes of the sperm. This results in an embyro with a total of 46 chromosomes (two pairs of 23).
An interesting phenomenon occurs during DNA replication in the first meiotic stage. At this time, pieces of DNA may shift between the matched chromosome pairs, in a process called crossing over. Crossing over increases the genetic variability of the offspring, and is one reason why siblings within a family may vary considerably in genotype and phenotype.
Control of Cellular Replication and Division
Some cells, such as liver, bone marrow, and gut cells, undergo replication and mitosis frequently. Other cells, such as nerve and cardiac muscle cells, do not replicate or divide except during fetal development or in the neonatal period. Growth factors, hormones, and other cell products turn cell division on or off and determine whether and how frequently a cell will replicate and divide. These factors may affect the replication and division of the cell that produces them, or they may circulate and affect a different cell. Physical factors such as crowding can also influence cell division.
● Protein Synthesis
Protein synthesis is an ongoing process in all cells. Protein synthesis occurs when sections of DNA are turned on, which causes the cell to begin making a certain protein. Although each cell contains identical DNA on the 46 chromosomes, some cells have different sections of DNA turned on at a given time compared to other cells. Sections of DNA that turn on and off in different cells are called genes. There are approximately 20,000 to 40,000 genes in the human body distributed among the 46 chromosomes. Each gene contains 90 to 3000 DNA molecules. Each gene codes for a particular protein or enzyme. Turning on or off different genes causes a cell to make different proteins compared to other cells.
Although genes controlling protein synthesis are present in the nucleus, proteins are made in the cytoplasm on specialized structures called ribosomes. The message from the activated gene in the nucleus must be carried to the ribosomes. This is accomplished by making a copy of the gene in the nucleus and transporting the copy to the ribosomes, where it is then translated into a protein.
TRANSCRIPTION OF DNA INTO MESSENGER RNA
To transcribe or make a copy of a gene, the area of the double helix on the chromosome where that gene is contained must unravel. Once unraveled, a special enzyme, called an RNA polymerase, attaches to a certain section at the start of the gene, called the promoter or controlling sequence. When the RNA polymerase attaches to this site, the gene is copied as a mirror image, in a manner similar to that described for DNA replication. The product is a similar molecule called ribonucleic acid (RNA). RNA, like DNA, contains phosphoric acid, but unlike DNA, it contains the sugar ribose instead of deoxyribose and the base uracil instead of thymine. When the gene is copied as RNA, each cytosine base in the gene becomes a guanine in the copy, each guanine becomes a cytosine, each thymine becomes an adenine, and each adenine becomes a uracil. The entire gene is transcribed by this procedure. After the gene is copied, the RNA polymerase will reach a special sequence on the DNA (called the termination sequence) and the process will stop. The RNA copy is released from the gene and moves into the cytoplasm. The RNA copy that carries the DNA message out of the nucleus is called the messenger RNA (mRNA). The mRNA then moves through the cytoplasm to the ribosomes (Fig. 2-5).
Adenine, guanine, cytosine, and uracil are carried as mRNA to the ribosomes in groups of three, called triplets or codons. Each triplet codes for one amino acid. There are 20 amino acids used in the human body that combine in various ways to make up all the proteins of the body. The long strand of mRNA triplets can be snipped at any point before the molecule leaves the nucleus, allowing different proteins to be made from one original gene.
TRANSFER RNA
Before the ribosomes make the protein from the mRNA template, another type of RNA, called transfer RNA (tRNA), binds to the mRNA by connecting mirror-image bases (called anticodons) to each triplet of mRNA bases. At the opposite end of the anticodon is the amino acid coded for by those three bases. There are at least 20 types of tRNA, each one carrying a certain amino acid on one end and the anticodon for that amino acid on the other end.
THE TRANSLATION OF MESSENGER RNA INTO PROTEIN
When the mRNA has found its matching tRNA, both molecules bind onto the ribosome, which is composed partly of a third type of RNA—ribosomal RNA. The amino acid carried by the tRNA is added to a chain of amino acids growing on the ribosome until the ribosome is signaled to stop adding to the chain by a special codon known as a stop codon. The protein is then complete and is freed from the ribosome.
CONTROL OF PROTEIN SYNTHESIS
Regulatory proteins block or activate the promoter section of each gene in the cell, determining which genes will be turned on, transcribed into mRNA, and made into a protein. If a regulatory protein blocks the promoter region of a gene, protein synthesis will not occur from that gene. If a regulatory protein binds to, or near, the promoter area in such a way that it makes the area accessible to the RNA polymerase, it will activate the gene’s transcription into mRNA. This type of protein would be considered a transcription factor or enhancer; if, on the other hand, a regulatory protein blocked the promoter area of a gene so that it was not transcribed into mRNA, the regulator protein would be a repressor.
Production and activation of regulatory proteins appear to be linked to other genes responding to feedback signals, chemical cues, and hormones such as thyroid hormone and growth hormone. These signals result in the production of proteins with repressor or activator functions. Other factors that alter the function of the histones responsible for folding and exposing different portions of the DNA may also affect DNA transcription. Methylation (adding a CH3
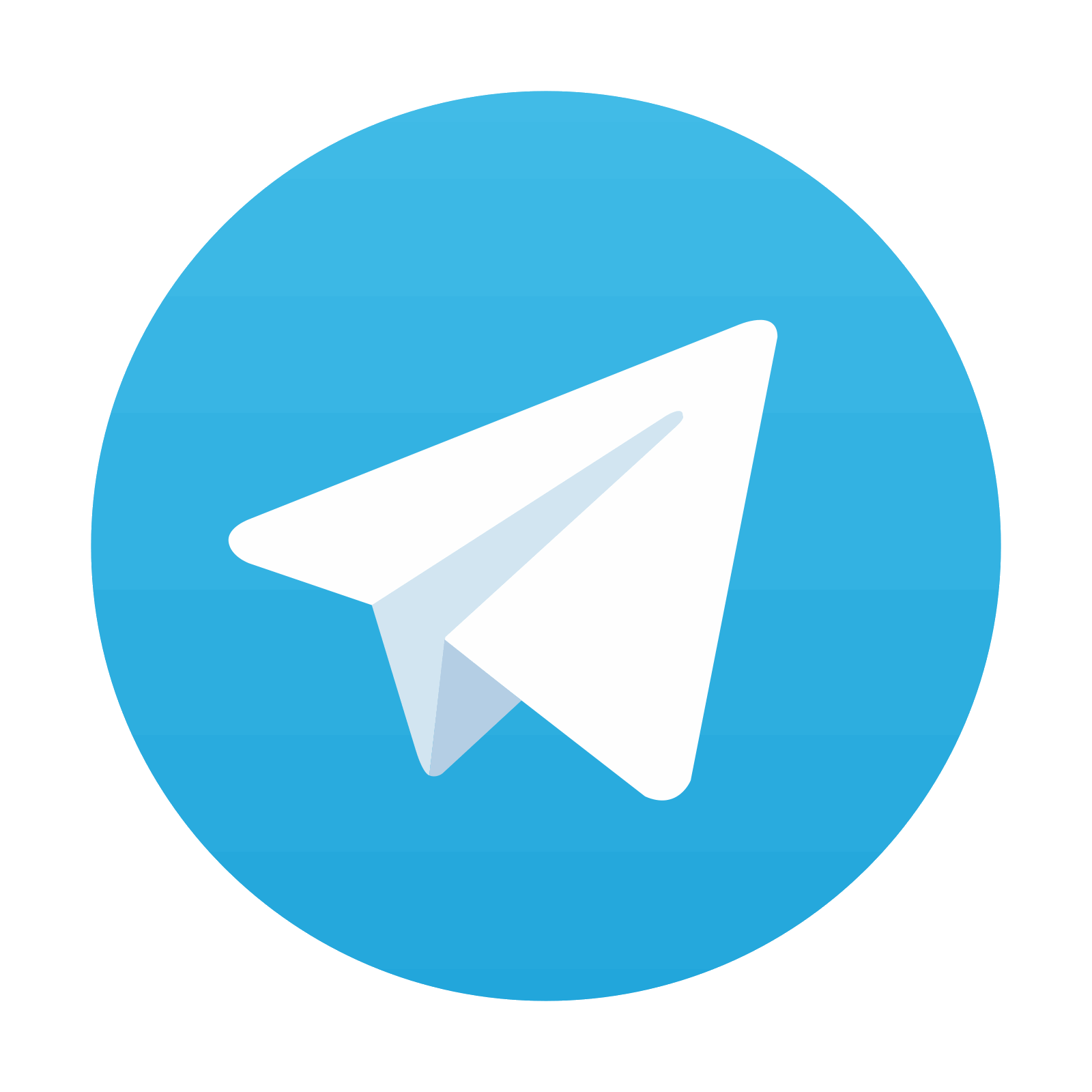
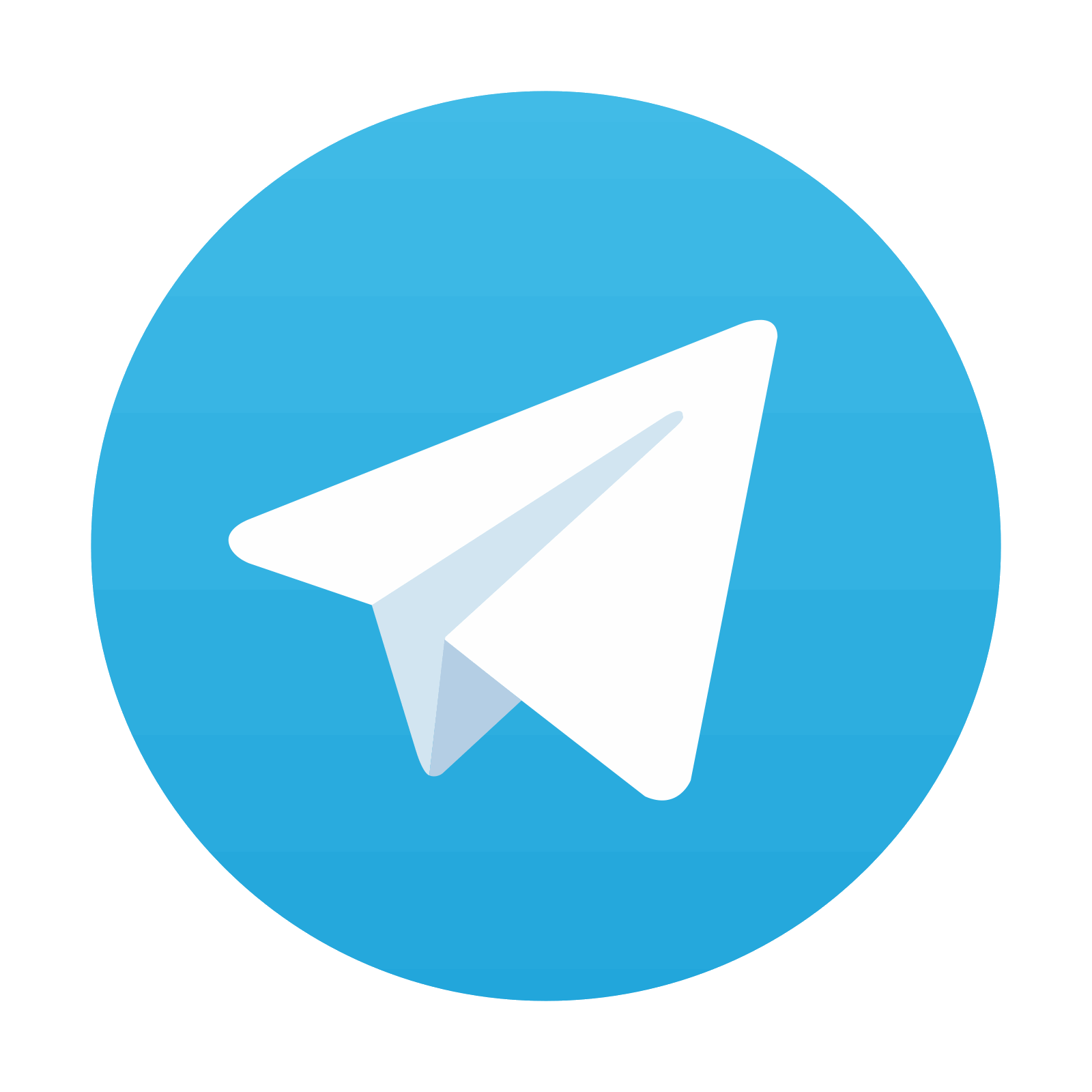
Stay updated, free articles. Join our Telegram channel
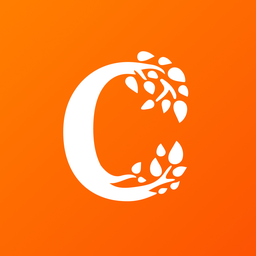
Full access? Get Clinical Tree
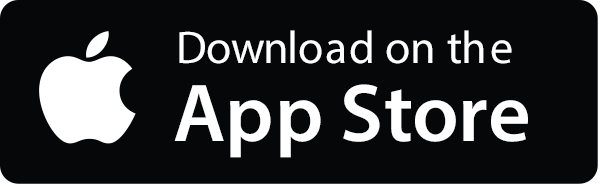
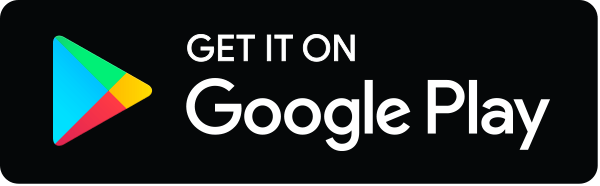
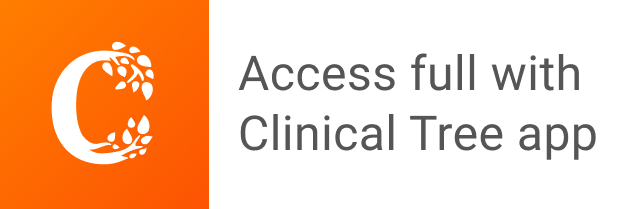