As we have seen in previous chapters, significant advances have occurred in many areas of medical genetics, including DNA sequencing technology, disease-gene identification, and cytogenetics. These developments have paved the way for more accurate and efficient testing of genetic disorders. Genetic testing can be defined as the analysis of chromosomes, DNA, RNA, proteins, or other analytes ∗
∗ An analyte is any substance that is subject to analysis.
to detect abnormalities that can cause a genetic disease. Indications for genetic testing include prenatal diagnosis, heterozygote carrier detection, and presymptomatic diagnosis of genetic disease. The principles and applications of genetic testing in these contexts are one focus of this chapter.The other focus is the treatment of genetic disease. Many aspects of disease management involve areas of medicine, such as surgery and drug treatment, which are beyond the scope of this book. However, gene therapy, in which patients’ cells are genetically altered to combat specific diseases, is being used increasingly to treat disease and is discussed in the second part of this chapter.
Population Screening for Genetic Disease
Screening tests represent an important component of routine health care. These tests are usually designed to detect treatable human diseases in their presymptomatic stage. Papanicolaou (Pap) tests for detecting cervical dysplasia and population screening for hypercholesterolemia are well-known examples of this public health strategy. Population screening is large-scale testing of populations for disease in an effort to identify persons who probably have the disease and those who probably do not. Screening tests are not intended to provide definitive diagnoses; rather, they are aimed at identifying a subset of the population on whom further, more exact, diagnostic tests should be carried out. This important distinction is commonly misunderstood by the lay public and seldom clarified by the popular media.
Genetic screening is population screening for genetic variants that can cause the disease in the person carrying the variant or in the descendants of the carrier. Newborn screening for inherited metabolic diseases (see Chapter 7 ) is a good example of the first type of genetic screening, and heterozygote detection for Tay–Sachs disease (discussed later) exemplifies the second. These two examples involve screening of populations, but genetic screening can also be applied to members of families with a positive history of a genetic condition. An example is testing for a balanced reciprocal translocation in families in which one or more members have had a chromosome disorder (see Chapter 6 ). Box 13.1 lists the various types of genetic screening, including several forms of prenatal diagnosis that are discussed in this chapter.
The goal of screening is early recognition of a disorder so that intervention will prevent or reverse the disease process (as in newborn screening for inborn errors of metabolism), or so that informed reproductive decisions can be made (as in screening for heterozygous carriers of an autosomal recessive mutation). A positive result from a genetic screening test is typically followed by a more precise diagnostic test.
Population Screening for Genetic Disorders
Newborn Screening
Blood
- ▪
Phenylketonuria, all 50 states in the United States
- ▪
Galactosemia, all 50 states in the United States
- ▪
Hypothyroidism, all 50 states in the United States
- ▪
Hemoglobinopathies, nearly all states
- ▪
Other: cystic fibrosis, congenital adrenal hyperplasia, maple syrup urine disease, homocystinuria, tyrosinemia, and several other diseases or inborn errors of metabolism are screened in the US and Europe
- ▪
Universal newborn hearing screening (>60% of congenital hearing loss is due to genetic factors)
Heterozygote Screening
Tay-Sachs disease, Ashkenazi Jewish population
Sickle cell disease, African-American population
Thalassemias in at-risk ethnic groups
Cystic fibrosis in some populations (persons of European descent, Ashkenazi Jews)
Prenatal Diagnosis of Genetic Disorders
Diagnostic Testing (Invasive Prenatal Diagnosis)
Amniocentesis
Chorionic villus sampling
Percutaneous umbilical blood sampling (PUBS)
Preimplantation genetic diagnosis
Fetal Visualization Techniques
Ultrasonography
Radiography
Magnetic resonance imaging
Population Screening
Maternal age >35 years
Family history of condition diagnosable by prenatal techniques
Quadruple screen: maternal serum α-fetoprotein, estriol, human chorionic gonadotropin, inhibin-A
First trimester screening: ultrasonography, PAPP-A, and free β subunit of human chorionic gonadotropin
Noninvasive prenatal screening using cell-free DNA from maternal plasma
Family Screening for Genetic Disorders
Family history of chromosomal rearrangement (e.g., translocation)
Screening female relatives in an X-linked pedigree (e.g., Duchenne muscular dystrophy, fragile X syndrome)
Heterozygote screening within at-risk families (e.g., cystic fibrosis)
Presymptomatic screening (e.g., Huntington disease, breast cancer, colon cancer)
Principles of Screening
The basic principles of screening were developed in the 1960s and are still widely utilized in decision making about initiating a new screening program. Characteristics of the disease, the test, and the health-care system should be considered when deciding whether population screening is appropriate for a particular disease.
First, the condition should be serious and relatively common. This ensures that the benefits to be derived from the screening program will justify its costs. The natural history of the disease should be clearly understood. There should be an acceptable and effective treatment, or in the case of some genetic conditions, prenatal diagnosis should be available. As for the screening test itself, it should be acceptable to the population, easy to perform, and relatively inexpensive. The screening test should be valid and reliable. Finally, the resources for diagnosis and treatment of the disorder must be accessible. A strategy for communicating results efficiently and effectively must be in place.
Screening programs typically use tests that are widely applicable and inexpensive to identify an at-risk population (e.g., the phenylketonuria (PKU) screening program, discussed in Clinical Commentary 13.1 ). Members of this population are then targeted for subsequent tests that are more accurate, but also more expensive and time consuming. In this context, the screening test should be able to effectively separate persons who have the disease from those who do not. This attribute, which defines the test’s validity, involves two components: sensitivity and specificity. Sensitivity reflects the ability of the test to correctly identify those with the disease. It is measured as the fraction of affected persons in whom the test is positive (i.e., true positives ). Specificity is the ability of the test to correctly identify those without the disease. It is measured as the fraction of unaffected persons in whom the test is negative (i.e., true negatives ). Sensitivity and specificity are determined by comparing the screening results with those of a definitive diagnostic test ( Table 13.1 ).
Disease Characteristics
Population screening of newborns for PKU represents an excellent example of the application of the screening model to genetic disease. As discussed in Chapter 4 , the prevalence of this autosomal recessive disorder of phenylalanine metabolism is about 1 in 10,000 to 15,000 white births in the United States. The natural history of PKU is well understood. More than 95% of untreated PKU patients develop moderate to severe intellectual disability. The condition is difficult to diagnose clinically in the first year of life because the physical signs are subtle and PKU usually manifests only as developmental delay. Dietary restriction of phenylalanine, when begun before 4 weeks of age, is highly effective in altering the course of the disease. A low-phenylalanine diet, although not particularly palatable, largely eliminates the IQ loss that would otherwise occur (an important exception is those who have a defect in biopterin metabolism, in whom a different therapy is used).
Test Characteristics
Until recently PKU was typically detected by the measurement of blood phenylalanine using a bacterial inhibition assay, the Guthrie test. Blood is collected in the newborn period, usually by heel stick, and placed on filter paper. The dried blood is placed on an agar plate and incubated with a strain of bacteria (Bacillus subtilis) that requires phenylalanine for growth. Measurement of bacterial growth permits quantification of the amount of phenylalanine in the blood sample. Tandem mass spectrometry (see below) is now usually used to screen for PKU and a number of other inborn errors of metabolism. Positive test results are repeated and followed by a quantitative assay of plasma phenylalanine and tyrosine.
If the test is performed after 2 days of age and after regular feeding on a protein diet, the detection rate (sensitivity) is about 98%. If it is performed at less than 24 hours of age, the sensitivity is about 84%, and for this reason a repeat test is recommended a few weeks after birth. Specificity is close to 100%.
System Characteristics
Because of the requirement of normal protein in the diet, many states request rescreening at 2 to 4 weeks of age. At that point, sensitivity approaches 100%. A high sensitivity level is important because of the severe impact of a missed diagnosis.
Phenylalanine levels in children with classic PKU typically exceed 20 mg/dL. For every 20 positive PKU screening results, only one infant has classic PKU (thus the positive predictive value is 5%—see below.). The others represent either false-positive findings (usually due to a transient reversible tyrosinemia) or infants with a form of hyperphenylalaninemia (elevated phenylalanine) not caused by classic PKU.
The cost of PKU screening is typically less than a few dollars per test. Several studies have shown that the cost of nationwide PKU screening is significantly less than the savings it achieves by avoiding care costs and lost productivity.
These principles apply to many of the other inborn errors that are now screened for in the expanded newborn screening programs in North America and Europe (see Chapter 7 ).
Actual Disease State | ||
---|---|---|
Result of Screening Test | Affected | Unaffected |
Test positive (+) | a (true positives) | b (false positives) |
Test negative (–) | c (false negatives) | d (true negatives) |
∗ a, b, c, and d represent the number of individuals in a population who were found to have each possible combination of test results and disease states. The test’s sensitivity = a/(a + c); specificity = d/(b + d); positive predictive value = a/(a + b); and negative predictive value = d/(c + d).
Screening tests are seldom, if ever, 100% sensitive and 100% specific. This is because the range of test values in the disease population overlaps that of the unaffected population ( Fig. 13.1 ). Thus results of a screening test (as opposed to the definitive follow-up diagnostic test) will be incorrect for some members of the population. Usually a cutoff value is designated to separate the diseased and nondiseased portions of the population. A trade-off exists between the impact of nondetection or low sensitivity (i.e., an increased false-negative rate) and the impact of low specificity (an increased false-positive rate). If the penalty for missing affected persons is high (as in untreated PKU), then the cutoff level is lowered so that nearly all disease cases will be detected (higher sensitivity). This also lowers the specificity by increasing the number of unaffected persons with positive test results (false positives) who are targeted for subsequent diagnostic testing. If confirmation of a positive test is expensive or hazardous, then false-positive rates are minimized (i.e., the cutoff level is increased, producing high specificity at the expense of sensitivity).
The basic elements of a test’s validity include its sensitivity (proportion of true positives accurately detected) and specificity (proportion of true negatives accurately detected). When sensitivity is increased, specificity decreases, and vice versa.

A primary concern in the clinical setting is the accuracy of a positive screening test. One needs to know the fraction of persons with a positive test result who truly have the disease in question [i.e., a/(a + b) in Table 13.1 ]. This quantity is defined as the positive predictive value. It is also useful to know the negative predictive value, which is the fraction of persons with a negative result who truly do not have the disease [d/(c + d)].
The concepts of sensitivity, specificity, and positive predictive value can be illustrated by an example. Congenital adrenal hyperplasia (CAH) due to a deficiency of 21-hydroxylase is an inborn error of steroid biosynthesis that can produce ambiguous genitalia in females and adrenal crises in both sexes. The screening test, a 17-hydroxyprogesterone assay, has a sensitivity of about 95% and a specificity of 99% ( Table 13.2 ). The prevalence of CAH is about 1/10,000 in most white populations, but it rises to about 1/400 in the Yupik Native Alaskan population.
Result of Screening Test | CAH Present | CAH Absent |
---|---|---|
Positive | ||
White | 47 | 5000 |
Yupik | 24 | 100 |
Negative | ||
White | 3 | 494,950 |
Yupik | 1 | 9875 |
∗ White positive predictive value = 47/(47 + 5000) ≈ 1%; Yupik positive predictive value = 24/(24 + 100) ≈ 19%. CAH, congenital adrenal hyperplasia.
Let us assume that a screening program for CAH has been developed in both of these populations. In a population of 500,000 whites, the false-positive rate (1—specificity) is 1%. Thus about 5000 unaffected persons will have a positive test. With 95% sensitivity, 47 of the 50 white persons who have CAH will be detected through a positive test. Note that the great majority of people who have a positive test result would not have CAH: the positive predictive value is 47/(47 + 5000), or less than 1%. Now suppose that 10,000 members of the Yupik population are screened for CAH. As Table 13.2 shows, 24 of 25 persons with CAH will test positive, and 100 persons without CAH will also test positive. Here the positive predictive value is much higher than in the white population: 24/(24 + 100) = 19%. This example illustrates an important principle: For a given level of sensitivity and specificity, the positive predictive value of a test increases as the prevalence of the disease increases.
The positive predictive value of a screening test is defined as the percentage of positive tests that are true positives. It increases as the prevalence of the target disorder increases.
Newborn Screening for Inborn Errors of Metabolism
Newborn screening programs represent an ideal opportunity for presymptomatic detection and prevention of genetic disease. All states in the United States screen newborns for PKU, galactosemia (see Chapter 7 ), and hypothyroidism. These conditions all fulfill the previously stated criteria for population screening. Each is a disorder in which the person is at significant risk for intellectual disability, which can be prevented by early detection and effective intervention.
In recent years, most states of the United States and many other nations have instituted screening programs to identify neonates with hemoglobin disorders (e.g., sickle cell disease). These programs are justified by the fact that up to 15% of untreated children with sickle cell disease die from infections before 5 years of age (see Chapter 3 ). Effective treatment, in the form of prophylactic antibiotics, is available. Some communities have begun screening for Duchenne muscular dystrophy by measuring creatine kinase levels in newborns. The objective is not presymptomatic treatment; rather it is identification of families who should receive genetic counseling in order to make informed reproductive decisions.
Increasingly, tandem mass spectrometry is used to screen newborns for protein variants that signal amino acid disorders (e.g., PKU, tyrosinemia, homocystinuria), organic acid disorders, and fatty acid oxidation disorders (e.g., MCAD and LCHAD deficiencies; see Chapter 7 ). This method begins with a sample of material from a dried blood spot, which is subjected to analysis by two mass spectrometers. The first spectrometer separates ionized molecules according to their mass, and the molecules are fragmented. The second spectrometer assesses the mass and charge of these fragments, which allows a computer to generate a molecular profile of the sample. Tandem mass spectrometry is highly accurate and very rapid; more than 30 disorders can be screened in approximately 2 minutes.
As screening technologies have continued to advance, many countries have established expanded newborn screening programs to deal with positive results and to provide rapid treatment of verified disease conditions. The American College of Medical Genetics and Genomics has developed guidelines and action plans for all of the conditions that are currently screened ( https://www.acmg.net ), and is consistently developing new plans for disorders for which screening is being considered (e.g., Pompe disease). Conditions for which newborn screening is commonly performed are summarized in Table 13.3 .
Newborn screening is an effective public health strategy for treatable disorders such as PKU, hypothyroidism, galactosemia, and sickle cell disease. The use of tandem mass spectrometry has expanded the number of diseases that can be detected by newborn screening.
Disease | Inheritance | Prevalence | Screening Test | Treatment |
---|---|---|---|---|
Phenylketonuria | Autosomal recessive | 1/10,000–1/15,000 | Tandem mass spectrometry | Dietary restriction of phenylalanine |
Galactosemia | Autosomal recessive | 1/50,000–1/100,000 | Transferase assay | Dietary restriction of galactose |
Congenital hypothyroidism | Usually sporadic | 1/5000 | Measurement of thyroxine (T 4 ) or thyroid stimulating hormone (TSH) | Hormone replacement |
Sickle cell disease | Autosomal recessive | 1/400–1/600 blacks | Isoelectric focusing or DNA diagnosis | Prophylactic penicillin |
Cystic fibrosis | Autosomal recessive | 1/2500 | Immunoreactive trypsinogen confirmed by DNA diagnosis | Antibiotics, chest physical therapy, pancreatic enzyme replacement if needed |
Heterozygote Screening
The aforementioned principles of population screening can be applied to the detection of unaffected carriers of disease-causing mutations. The target population is a group known to be at risk. The intervention consists of the presentation of risk figures and options such as prenatal diagnosis. Genetic diseases amenable to heterozygote screening are typically autosomal recessive disorders for which prenatal diagnosis and genetic counseling are available, feasible, and accurate.
An example of a highly successful heterozygote screening effort is the Tay–Sachs screening program in North America. Infantile Tay–Sachs disease is an autosomal recessive lysosomal storage disorder in which the lysosomal enzyme β-hexosaminidase A (HEX A) is deficient (see Chapter 7 ), causing a buildup of the substrate, G M2 ganglioside, in neuronal lysosomes. The accumulation of this substrate damages the neurons and leads to blindness, seizures, hypotonia, and death by about 5 years of age. Tay–Sachs disease is especially common among Ashkenazi Jews, with a heterozygote frequency of about 1 in 30. Thus this population is a reasonable candidate for heterozygote screening. Accurate carrier testing is available (assays for HEX A or direct DNA testing for mutations). Because the disease is uniformly fatal, options such as pregnancy termination or artificial insemination by noncarrier donors are acceptable to many couples. A well-planned effort was made to educate members of the target population about risks, testing, and available options. As a result of screening, the number of Tay–Sachs disease births among Ashkenazi Jews in the United States and Canada declined by 90%, from 30 to 40 per year before 1970, to 3 to 5 per year in the 1990s, and to zero by 2003.
Beta thalassemia major, another serious autosomal recessive disorder, is especially common among many Mediterranean and South Asian populations (see Chapter 3 ). Effective carrier screening programs have produced a 75% decrease in the prevalence of newborns with this disorder in Greece, Cyprus, and Italy. Carrier screening is also possible for cystic fibrosis, another autosomal recessive disorder ( Clinical Commentary 13.2 ). Table 13.4 presents a list of selected conditions for which heterozygote screening programs have been developed in many countries.
Approximately 2,000 mutations have now been reported in the CFTR gene, and although some are benign variants, most can cause cystic fibrosis. It would be technologically impractical to test for all reported mutations in a population screening program. However, among the mutations that can cause cystic fibrosis (CF) in whites, about 70% are the three-base deletion termed F508del (see Chapter 4 ). In this population, carrier screening using PCR-based detection of F508del would detect approximately 90% of couples in which one or both are heterozygous carriers of this mutation (1–0.30 2 , where 0.30 2 represents the frequency of carrier couples in whom neither carries the F508del mutation). It is now common to test simultaneously for 23 of the most common CFTR mutations, which will detect approximately 85% of all CFTR mutations in persons of European descent (because mutation frequencies vary among populations, this figure is somewhat lower in other U.S. populations, such as African-Americans and Hispanics). Among whites 98% of couples, in which one or both members carry a CF mutation, would be recognized (i.e., 1–0.15 2 ), yielding a high level of sensitivity. Some tests include more than 100 known CFTR mutations, leading to higher sensitivity in most ethnic groups. The American College of Medical Genetics and Genomics and the American College of Obstetricians and Gynecologists recommend that couples who are planning a pregnancy, or who are currently pregnant, should be offered a screen for CF carrier status. Couples in which both parents are heterozygotes would define a subset of the population in which prenatal diagnosis for CF might be offered. Notably, some commercial laboratories now offer heterozygous screens for pregnant couples for more than 100 recessive conditions. Newborn screening for CF is carried out in all 50 U.S. states. The first screening test measures the immunoreactive trypsinogen (IRT) level, which is elevated in newborns with CF because of pancreatic damage. A positive IRT test is usually followed by confirmatory genetic testing.
Disease | Ethnic Group | Carrier Frequency | At-Risk Couple Frequency | Disease Incidence in Newborns |
---|---|---|---|---|
Sickle cell disease | Blacks | 1/12 | 1/150 | 1/600 |
Tay–Sachs disease | Ashkenazi Jews | 1/30 | 1/900 | 1/3600 |
β-Thalassemia | Greeks, Italians | 1/30 | 1/900 | 1/3600 |
α-Thalassemia | Southeast Asians, Chinese | 1/25 | 1/625 | 1/2500 |
Cystic fibrosis | Northern Europeans | 1/25 | 1/625 | 1/2500 |
Phenylketonuria | Northern Europeans | 1/50 | 1/2500 | 1/10,000 |
Spinal muscular atrophy | Asians | 1/50 | 1/2500 | 1/10,000 |
In addition to the criteria for establishing a population screening program for genetic disorders, guidelines have been developed regarding the ethical and legal aspects of heterozygote screening programs. These are summarized in Box 13.2 .
Heterozygote screening consists of testing (at the phenotype or genotype level) a target population to identify unaffected carriers of a disease gene. The carriers can then be given information about risks and reproductive options.
Recommended guidelines:
Screening should be voluntary, and confidentiality must be ensured
Screening requires informed consent
Providers of screening services have an obligation to ensure that adequate education and counseling are included in the program
Quality control of all aspects of the laboratory testing, including systematic proficiency testing, is required and should be implemented as soon as possible
There should be equal access to testing
Presymptomatic Diagnosis
With the development of genetic diagnosis by direct mutation detection, presymptomatic diagnosis has become feasible for many genetic diseases. At-risk persons can be tested to determine whether they have inherited a disease-causing mutation before they develop clinical symptoms of the disorder. Presymptomatic diagnosis is available, for example, for Huntington disease, adult polycystic kidney disease, and autosomal dominant breast/ovarian cancer. By informing persons that they do or do not carry a disease-causing mutation, presymptomatic diagnosis can aid in making reproductive decisions. It can provide reassurance to those who learn that they do not carry a disease-causing mutation. In some cases, early diagnosis can improve health supervision. For example, persons who inherit an autosomal dominant breast cancer mutation can undergo mammography at an earlier age to increase the chances of early tumor detection. Persons at risk for the inheritance of RET mutations (see Chapter 11 ), who are highly likely to develop multiple endocrine neoplasia type 2 (MEN2), can undergo a prophylactic thyroidectomy to reduce their chance of developing a malignancy. Those who inherit mutations that cause some forms of familial colon cancer (adenomatous polyposis coli [APC] and hereditary nonpolyposis colorectal cancer [HNPCC]; see Chapter 11 ) can also benefit from early diagnosis and treatment.
Because most genetic diseases are relatively uncommon in the general population, universal presymptomatic screening is currently impractical. It is usually recommended only for persons who are known to be at risk for the disease, generally because of a positive family history.
Genetic testing can sometimes be performed to identify persons who have inherited a disease-causing gene before they develop symptoms. This is termed presymptomatic diagnosis.
Psychosocial Implications of Genetic Screening and Diagnosis
Screening for genetic diseases has many social and psychological implications. The burden of anxiety, cost, and potential stigmatization surrounding a positive test result must be weighed against the need for detection. Often screening tests are misperceived as diagnostically definitive. The concept that a positive screening test does not necessarily indicate disease presence must be emphasized to those who undergo screening (see Box 13.2 ).
The initial screening programs for sickle cell carrier status in the 1970s were plagued by misunderstandings about the implications of carrier status. Occasionally, carrier detection led to cancellation of health insurance or employer discrimination. Such experiences underscore the need for effective genetic counseling and public education. Other issues include the right to choose not to be tested and the potential for invasion of privacy.
The social, psychological, and ethical aspects of genetic screening will become more complicated as newer techniques of DNA diagnosis become more accessible. For example, even though presymptomatic diagnosis of Huntington disease is available, several studies have shown that fewer than 20% of at-risk persons elected this option. Largely, this reflects the fact that no effective treatment is currently available for this disorder. Presymptomatic diagnosis of BRCA1 and BRCA2 (see Chapter 11 ) carrier status has also met with mixed responses. In part, this has been a reaction to the cost of the test; because of the large number of different mutations in BRCA1 or BRCA2 that can cause breast or ovarian cancer, testing typically consists of sequencing all exons and promoters of both genes, as well as some intronic nucleotides near each exon. This has been a costly procedure, although new technologies and increasing competition are now decreasing the cost. Preventive measures such as prophylactic mastectomy and oophorectomy (removal of the breasts and ovaries, respectively) are known to reduce cancer risk by approximately 90%, but they do not completely eliminate it.
For some genetic diseases, such as autosomal dominant colon cancer syndromes, early diagnosis can lead to improved survival because effective preventive treatments are readily available (colectomy or polypectomy for precancerous colon polyps). In addition, many at-risk persons will find that they do not carry a disease-causing allele, allowing them to avoid unpleasant (and possibly hazardous) diagnostic procedures such as colonoscopy or mammography. However, as screening for such diseases becomes more common, the issues of privacy and confidentiality and the need for accurate communication of risk information must also be addressed.
Molecular Tools for Screening and Diagnosis
In the past, genetic screening usually relied on assays of the disease phenotype, such as a β-hexosaminidase assay for Tay–Sachs disease or a creatine kinase assay for Duchenne muscular dystrophy. Advances in DNA technology have led to diagnosis at the level of the genotype. In most cases direct assays of disease-causing mutations have been developed. Genetic diagnosis at the DNA level is now supplementing, and in many cases supplanting, tests based on phenotypic assays.
Linkage analysis and direct mutation diagnosis have been used for diagnostic testing within families for prenatal diagnosis of genetic disorders, and more recently, in population screening. Improved technology and an increased demand for testing have led to the establishment of clinical molecular laboratories in many medical centers throughout the world.
Linkage Analysis
DNA polymorphisms such as single nucleotide polymorphisms (SNPs) or short tandem repeats (STRs) can be used as markers in linkage analysis, as described in Chapter 8 . Once linkage phase is established in a family, the marker locus can be assayed to determine whether an at-risk person has inherited a chromosome segment that contains a disease-causing mutation or a homologous segment that contains a normal allele ( Fig. 13.2 ). Because this approach uses linked markers but does not involve direct examination of the disease-causing mutations, it is a form of indirect diagnosis.

Linkage analysis has been employed successfully in diagnosing many of the genetic diseases discussed in this text. In principle, it can be used to diagnose any mapped genetic disease. It has the advantages that the disease-causing gene and its product need not be known. The marker simply tells us whether or not the at-risk person has inherited the chromosome region that contains a disease-causing mutation.
The disadvantages of this approach are that several family members must be tested in order to establish linkage phase; not all markers are informative (sufficiently heterozygous) in all families (see Chapter 8 for a discussion of uninformative families); and recombination can occur between the marker and the disease-causing mutation, introducing a source of diagnostic error. With advances in direct mutation detection, indirect diagnosis with linked markers is applied less commonly than in the past, but it is still useful when a disease-causing mutation is being transmitted in a family but cannot be directly identified.
Linkage analysis, a form of indirect genetic diagnosis, uses linked markers to determine whether a person has inherited a chromosome containing a disease gene from his or her parent. The need for typing of numerous family members, and the possibilities of recombination and uninformative matings are disadvantages of this approach.
Direct Mutation Analysis
Direct diagnosis, in which the disease-causing mutation itself is detected, has become the approach used most commonly in genetic diagnosis. Compared to indirect diagnosis, it has the advantages that family information is not needed (the mutation is viewed directly in each individual), uninformative matings are not a problem, and there is no error resulting from recombination. ( Table 13.5 summarizes the advantages and disadvantages of direct and indirect diagnosis.) Several forms of direct diagnosis ( Table 13.6 ) have been described in preceding chapters. For example, fluorescence in situ hybridization (FISH) or array comparative genomic hybridization (array CGH) can be used to detect deletions or duplications of chromosome regions (see Chapter 6 ). Southern blotting or multiplex ligation-dependent probe amplification (MLPA) can be used to detect copy number variants or large repeat expansions (see Chapter 3 ).
Attribute | Indirect Diagnosis | Direct Diagnosis |
---|---|---|
Family information needed | Yes | No |
Errors possible because of recombination | Yes | No |
Markers may be uninformative | Yes | No |
Single test can uncover multiple mutations | Yes | Yes (by DNA sequencing) |
Disease-causing mutation must be identified | No | Yes |
Method | Single-Gene Mutations | Copy Number Variants (Including Deletions and Duplications) | Uniparental Disomy | Repeat Expansions | Examples of Applications |
---|---|---|---|---|---|
FISH | X | Prader-Willi syndrome; Williams syndrome; aneuploidy | |||
Array CGH or cytogenomic microarray | X | X | Intellectual disability or other conditions of unknown etiology; suspected microdeletions, microduplications, or aneuploidy | ||
SNP microarrays | X | X | Drug sensitivity; intellectual disability; cardiovascular disease risk | ||
Allele-specific oligonucleotide (ASO) hybridization | X | X | Sickle cell disease; α 1 -antitrypsin deficiency | ||
Sanger DNA sequencing | X | Familial breast/ovarian cancer | |||
Southern blot or MLPA | X | X | Myotonic dystrophy; fragile X syndrome | ||
Targeted disease-sequencing panels | X | Familial cardiomyopathy; long QT syndrome; familial colorectal cancer | |||
Whole-exome or whole-genome sequencing | X | X | Undiagnosed conditions or diseases of uncertain etiology |
∗ Adapted from Katsanis SH, Katsanis N. Molecular genetic testing and the future of clinical genomics. Nat Rev Genet . 2013;14:415–426.
If the DNA sequence surrounding a mutation is known, the region containing the sequence can be amplified by polymerase chain reaction (PCR) (see Chapter 3 ) and an oligonucleotide probe can be synthesized that will hybridize (undergo complementary base pairing) only to the mutated sequence (such probes are often termed allele-specific oligonucleotides, or ASOs). A second probe that will hybridize to the normal DNA sequence is also synthesized. Stringent hybridization conditions are used so that a one-base mismatch will prevent hybridization. DNA from persons who are homozygous for the mutation, hybridizes only with the ASO containing the mutated sequence, whereas DNA from persons homozygous for the normal sequence hybridizes with the normal ASO. DNA from heterozygotes hybridizes with both probes ( Fig. 13.3 ). The length of the ASO probes, usually about 18 to 20 nucleotides, is critical. Shorter probes would not be unique in the genome and would therefore hybridize to multiple regions. Longer probes are more difficult to synthesize correctly and could hybridize to both the normal and the mutated sequence.

Genetic diagnosis using ASO probes requires that the disease-causing gene has been identified and sequenced. In addition, each disease-causing mutation requires a different oligonucleotide probe. For this reason, although this approach is powerful, it can become more challenging if the disease may be caused by a large number of different mutations that each have low frequency in the population.
Direct genetic diagnosis is accomplished by assaying the disease-causing mutation itself. It is potentially more accurate than indirect diagnosis and does not require family information. Direct diagnosis can be performed by hybridization of a person’s DNA with allele-specific oligonucleotide probes. This approach is feasible if the DNA sequence causing a genetic disease is known and if the number of disease-causing mutations is limited.
Examples of diseases caused by a limited number of mutations include sickle cell disease and α 1 -antitrypsin deficiency ( Clinical Commentary 13.3 ). Although approximately 2,000 cystic fibrosis mutations have been identified, 23 of the most common ones account for the great majority of mutations in many populations (see Clinical Commentary 13.2 ). Thus direct diagnosis can be used to identify most cystic fibrosis homozygotes and heterozygous carriers. Prenatal diagnosis is also possible. Direct diagnosis can also be used to detect deletions or duplications (e.g., those of the dystrophin gene that cause most cases of Duchenne muscular dystrophy). Currently clinical genetic testing is available for more than 11,000 conditions, many of which are single-gene diseases. Tests are available for almost all of the single-gene conditions discussed in this textbook. Despite this wide availability, it should be kept in mind that genetic testing, like all testing procedures, has a number of limitations ( Box 13.3 ).
An inherited deficiency of α 1 -antitrypsin (AAT) is one of the most common autosomal recessive disorders among whites, affecting approximately 1 in 2000 to 1 in 5000. AAT, synthesized primarily in the liver, is a serine protease inhibitor. As its name suggests, it binds trypsin. However, AAT binds much more strongly to neutrophil elastase, a protease that is produced by neutrophils (a type of leukocyte) in response to infections and irritants. It carries out its binding and inhibitory role primarily in the lower respiratory tract, where it prevents neutrophil elastase from digesting the alveolar septa of the lung.
Persons with less than 10% to 15% of the normal level of AAT activity experience significant lung damage and typically develop emphysema during their 30s, 40s, or 50s. In addition, at least 10% develop liver cirrhosis as a result of the accumulation of variant AAT molecules in the liver. AAT deficiency accounts for almost 20% of all nonalcoholic liver cirrhosis in the United States. Cigarette smokers with AAT deficiency develop emphysema much earlier than do nonsmokers because cigarette smoke irritates lung tissue, increasing secretion of neutrophil elastase. At the same time, it inactivates AAT, so there is also less inhibition of elastase. One study showed that the median age of death of nonsmokers with AAT deficiency was 62 years, whereas it was 40 years for smokers with this disease. The combination of cigarette smoking (an environmental factor) and an AAT mutation (a genetic factor) produces more-severe disease than either factor alone; thus this is an example of a gene–environment interaction.
Typically, AAT deficiency is tested first by a straightforward assay for reduced serum AAT concentration. Because a variety of conditions can reduce serum AAT, additional testing, via a type of protein electrophoresis (see Chapter 3 ) or DNA testing, is carried out to confirm a diagnosis of AAT deficiency. Direct DNA testing became feasible with the identification of SERPINA1, the gene that encodes AAT. More than 120 SERPINA1 mutations have been identified, but only two missense variants, labeled the S and Z alleles, are common and clinically significant. Approximately ninety-five percent of cases of AAT deficiency are either ZZ homozygotes or SZ compound heterozygotes. The latter genotype generally produces less severe disease symptoms. Two large studies have indicated that the risk of developing emphysema among ZZ homozygotes is 70% for nonsmokers and 90% for smokers. Because the great majority of AAT cases are caused by two alleles, Z and S, this disease can be diagnosed efficiently and with 95% sensitivity by allele-specific DNA testing. If necessary, other disease-causing mutations can be detected by sequencing the entire gene.
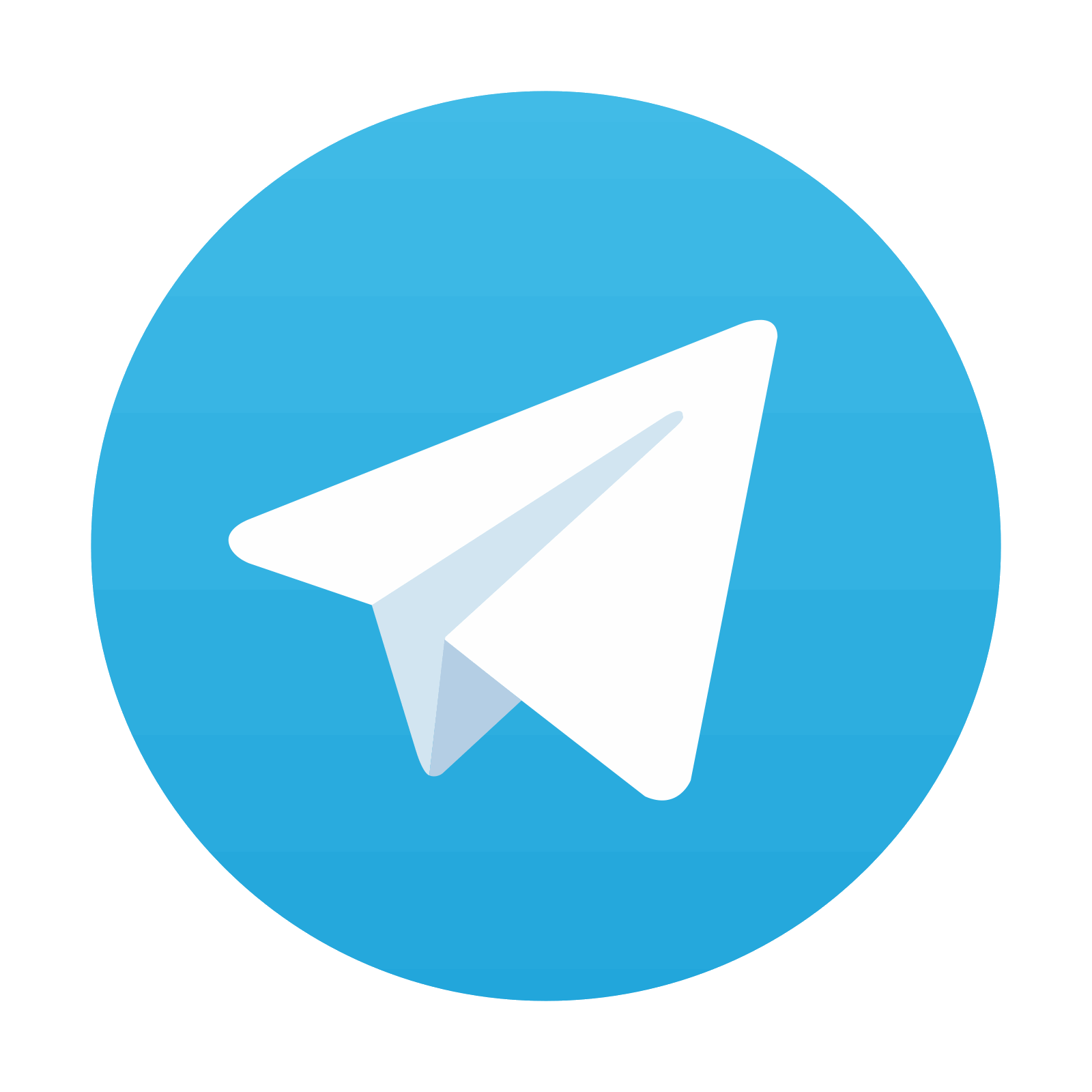
Stay updated, free articles. Join our Telegram channel
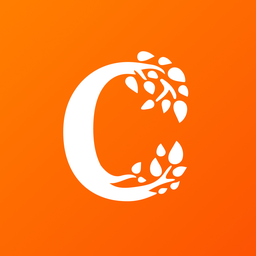
Full access? Get Clinical Tree
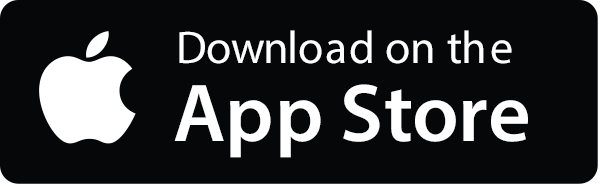
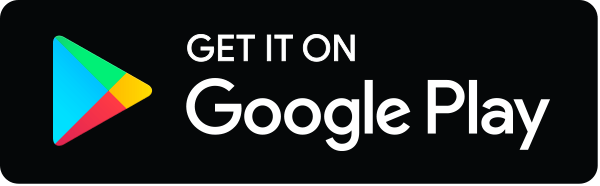
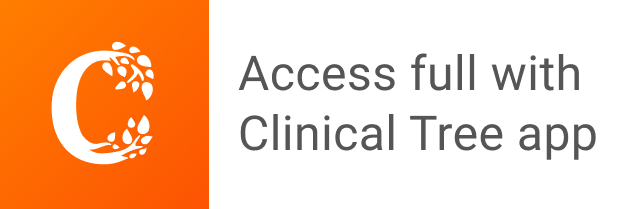