The previous chapter dealt with diseases that are inherited in patterns that were first elucidated by Gregor Mendel. In this chapter we discuss disease-causing mutations that are inherited in ways that were unknown to Mendel and are thus sometimes termed nonmendelian.
The first mutations to be discussed are DNA variants of the sex chromosomes (X and Y), known as sex-linked mutations. The human X chromosome is large, containing about 5% of the nuclear genome’s DNA (approximately 155 million base pairs [155 megabases, 155 Mb]). Nearly 1300 genes have been localized to the X chromosome, and the diseases caused by these genes are said to be X-linked. In contrast to the X chromosome, the Y chromosome is quite small (60 Mb) and contains only a few dozen genes.
Another group of disease-causing mutations is located in the mitochondrial genome, which is inherited only from one’s mother. Mitochondrial diseases thus display a unique pattern of inheritance in families. Extensive analyses have revealed a growing number of disease-causing mutations in the mitochondrial genome.
Finally, we discuss two processes that have been elucidated only in the past two to three decades: anticipation and imprinting. Anticipation refers to earlier age-of-onset of some genetic diseases in more recent generations of families. Imprinting refers to the fact that some genes are expressed only on paternally transmitted chromosomes and others are expressed only on maternally transmitted chromosomes. Our understanding of both of these processes has been greatly enhanced by detailed molecular analyses of humans and model organisms.
X
Inactivation
The X chromosome contains many important protein-coding genes, and it has long been known that human females have two X chromosomes and males have only one. Thus females have two copies of each X-linked gene, and males have only one copy. Yet males and females do not differ in terms of the amounts of protein products (e.g., enzyme levels) encoded by most of these genes. What could account for this?
In the early 1960s Mary Lyon hypothesized that one X chromosome in each somatic cell of the female is inactivated. This would result in dosage compensation, an equalization of the amount of X-linked gene products in males and females. The Lyon hypothesis stated that X inactivation occurs early in female embryonic development and that the X chromosome contributed by the father is inactivated in some cells, whereas in other cells the X chromosome contributed by the mother is inactivated. In each cell, one of the two X chromosomes is chosen at random for inactivation, so the maternally and paternally transmitted X chromosomes are each inactivated in about half of the embryo’s cells. Inactivation, like gamete transmission, is analogous to a coin-tossing experiment. Once an X chromosome is inactivated in a cell, it will remain inactive in all descendants of that cell. X inactivation is therefore a randomly determined, but fixed (or permanent) process. As a result of X inactivation, all normal females have two distinct populations of cells: one population has an active paternally derived X chromosome, and the other has an active maternally derived X chromosome. ( Fig. 5.1 provides a summary of this process.) Because they have two populations of cells, females are mosaics (see Chapter 4 ) for X chromosome activity. Males, having only one copy of the X chromosome, are not mosaics but are hemizygous for the X chromosome ( hemi means “half”).
The Lyon hypothesis states that one X chromosome in each cell is randomly inactivated early in the embryonic development of females. This ensures that females, who have two copies of the X chromosome, will produce X-linked gene products in quantities roughly similar to those produced in males (dosage compensation).

The Lyon hypothesis relied on several pieces of evidence, most of which were derived from animal studies. First, it was known that females are typically mosaics for some X-linked traits and males are not. For example, female “calico” cats have alternating black and orange patches of fur that correspond to two populations of cells: one that contains X chromosomes in which an “orange” allele is active and one that contains X chromosomes in which a “black” allele is active. Male cats of this breed do not exhibit alternating colors and have either black or orange patches of fur. Another example seen in humans is X-linked ocular albinism. This is an X-linked recessive condition characterized by a lack of melanin production in the retina and by ocular problems such as nystagmus (rapid involuntary eye movements) and decreased visual acuity. Males who inherit the mutation show a relatively uniform lack of melanin in their retinas, whereas female heterozygotes exhibit alternating patches of pigmented and nonpigmented tissue ( Fig. 5.2 ).

The Lyon hypothesis was also supported by biochemical evidence. The enzyme glucose-6-phosphate dehydrogenase (G6PD) is encoded by a gene on the X chromosome and is present in equal quantities in males and females (dosage compensation). In females who are heterozygous for two common G6PD alleles (labeled A and B ), some skin cells produce only the A variant of the enzyme and others produce only the B variant. This is further proof of X chromosome mosaicism in females.
Finally, cytogenetic studies in the 1940s showed that interphase cells of female cats often contained a densely staining chromatin mass in their nuclei. These masses were not seen in males. They were termed Barr bodies, after Murray Barr, one of the scientists who described them. Barr and his colleague Ewart Bertram hypothesized that the Barr body represented a highly condensed X chromosome. It is now known that Barr and Bertram were correct and that the inactive X chromosome is observable as a Barr body in the somatic cells of normal females. Its condensed state is correlated with reduced transcriptional activity, and its DNA is replicated later in the S phase than that of other chromosomes.
The Lyon hypothesis is supported by cytogenetic evidence: Barr bodies, which are inactive X chromosomes, are seen only in cells with two or more X chromosomes. It is also supported by biochemical and animal studies that reveal mosaicism of X-linked traits in female heterozygotes.
Further study has largely verified the Lyon hypothesis. Messenger RNA (mRNA) is transcribed from only one X chromosome in each somatic cell of a normal female. The inactivation process takes place within approximately 7 to 10 days after fertilization, when the embryonic inner-cell mass contains no more than a few dozen cells. Inactivation is initiated in a single 1-Mb region on the X chromosome long arm, the X inactivation center, and then spreads along the chromosome. Although inactivation is random among cells that make up the embryo itself, only the paternally derived X chromosome is inactivated in cells that will become extraembryonic tissue (e.g., the placenta). X inactivation is permanent for all somatic cells in the female, but the inactive X chromosome must later become reactivated in the female’s germline so that each of her egg cells will receive one active copy of the X chromosome.
An important implication of the Lyon hypothesis is that the number of Barr bodies in somatic cells is always one less than the number of X chromosomes. Normal females have one Barr body in each somatic cell, and normal males have none. Females with Turner syndrome (see Chapter 6 ), having only one X chromosome, have no Barr bodies. Males with Klinefelter syndrome (two X chromosomes and a Y chromosome) have one Barr body in their somatic cells, and females who have three X chromosomes per cell have two Barr bodies in each somatic cell. This pattern leads to another question: if the extra X chromosomes are inactivated, why aren’t people with extra (or missing) X chromosomes phenotypically unaffected?
The answer to this question is that X inactivation is incomplete. Some regions of the X chromosome remain active in all copies. For example, the tips of the short and long arms of the X chromosome do not undergo inactivation. The tip of the short arm of the X chromosome is homologous to the distal short arm of the Y chromosome (see Chapter 6 ). In total, about 15% to 20% of the genes on the human X chromosome escape inactivation, and relatively more genes on the short arm escape inactivation than on the long arm. Some of the X-linked genes that remain active on both copies of the X chromosome have homologs on the Y chromosome, preserving equal gene dosage in males and females. Thus having extra (or missing) copies of active portions of the X chromosome contributes to a disease phenotype.
X inactivation is random, fixed, and incomplete. The last feature helps to explain why, despite X inactivation, most persons with abnormal numbers of sex chromosomes have a disease phenotype.
The X inactivation center contains a gene, XIST, which is transcribed only on the inactive X chromosome; its 17-kb mRNA transcripts are detected in normal females but not in normal males. The RNA transcript, however, is not translated into a protein and is an example of a long noncoding RNA (lncRNA; see Chapter 2 ). The XIST RNA transcript remains in the nucleus and coats the inactive X chromosome, recruiting other cellular proteins that inhibit transcription. This process acts as a signal that leads to other aspects of inactivation, including late replication and condensation of the inactive X chromosome.
Methylation and histone deacetylation are additional features of the inactive X chromosome. Many CG dinucleotides in the 5′ regions of genes on the inactive X are heavily methylated, and the administration of demethylating agents, such as 5-azacytidine, can partially reactivate an inactive X chromosome in vitro. However, methylation does not appear to be involved in spreading the inactivation signal from the inactivation center to the remainder of the X chromosome. It is more likely to be responsible for maintaining the inactivation of a specific X chromosome in a cell and all of its descendants.
The XIST gene is located in the X inactivation center and is required for X inactivation. It encodes a lncRNA product that coats the inactive X chromosome. X inactivation is also associated with methylation of the inactive X chromosome, a process that might help to ensure the long-term stability of inactivation.
Sex-Linked Inheritance
Sex-linked genes are those that are located on either the X or the Y chromosome. Because only a few dozen genes are known to be located on the human Y chromosome, our attention will be focused mostly on X-linked diseases. These have traditionally been grouped into X-linked recessive and X-linked dominant categories, and these categories are used here for consistency with other literature. However, because of variable expression, incomplete penetrance, and the effects of random X inactivation, the distinction between X-linked dominant and X-linked recessive inheritance is sometimes ambiguous.
X-Linked Recessive Inheritance
A number of well-known diseases and traits are caused by X-linked recessive genes. These include hemophilia A ( Clinical Commentary 5.1 ), Duchenne muscular dystrophy ( Clinical Commentary 5.2 ), and red–green color blindness (see Box 5.1 ). Additional X-linked diseases are listed in Table 5.1 . The inheritance patterns and recurrence risks for X-linked recessive diseases differ substantially from those for diseases caused by autosomal genes.
From Hoffbrand VA. Color Atlas of Clinical Hematology. 3rd ed. Philadelphia: Mosby; 2000:281-283.
Modified from McCance K, Huether S. Pathophysiology: The Biologic Basis for Disease in Adults and Children. 5th ed. St. Louis: Mosby; 2005.
Hemophilia A is caused by mutations in the gene that encodes clotting factor VIII and affects approximately 1 in 5000 to 1 in 10,000 males worldwide. It is the most common of the severe bleeding disorders and has been recognized as a familial disorder for centuries. The Talmud states that boys whose brothers or cousins bled to death during circumcision are exempt from the procedure (this may well be the first recorded example of genetic counseling).
Hemophilia A is caused by deficient or defective factor VIII, a key component of the clotting cascade. Fibrin formation is affected, resulting in prolonged and often severe bleeding from wounds and hemorrhages in the joints and muscles ( Fig. 5.3 ). Bruising is often seen. Hemarthroses (bleeding into the joints) are common in the ankles, knees, hips, and elbows. These events are often painful, and repeated episodes can lead to destruction of the synovium and diminished joint function. Intracranial hemorrhages can occur and are a leading cause of death. Platelet activity is normal in hemophiliacs, so minor lacerations and abrasions do not usually lead to excessive bleeding.

Hemophilia A varies considerably in its severity, and this variation is correlated directly with the level of factor VIII. About half of hemophilia A patients fall into the severe category, with factor VIII levels that are less than 1% of normal. These persons experience relatively frequent bleeding episodes, often several per month. Patients with moderate hemophilia (1%–5% of normal factor VIII) generally have bleeding episodes only after mild trauma and typically experience one to several episodes per year. Persons with mild hemophilia have 5% to 30% of the normal factor VIII level and usually experience bleeding episodes only after surgery or relatively severe trauma.
Historically, hemophilia A was often fatal before 20 years of age, but a major advance in treatment came in the early 1960s with the ability to purify factor VIII from donor plasma. Factor VIII is usually administered at the first sign of a bleeding episode and is a highly effective treatment. Prophylactic factor VIII administration in severe hemophiliacs is effective in preventing loss of joint function. By the 1970s, the median age at death of persons with hemophilia had increased to 68 years.
The major drawback of donor-derived factor VIII was the fact that because a typical infusion contained plasma products from hundreds or thousands of different donors, it was often contaminated by viruses. Consequently, patients often suffered from hepatitis B and C infections. Even more seriously, human immunodeficiency virus (HIV) can be transmitted in this manner, and it is estimated that half of American hemophilia patients treated with donor-derived factor VIII between 1978 and 1985 became infected with HIV. From 1979 to 1998, acquired immune deficiency syndrome (AIDS) accounted for nearly half of deaths among Americans with hemophilia A, which resulted in a decrease in the median age at death to 49 years in the 1980s. Donor blood has been screened for HIV since 1985, and heat treatment of donor-derived factor VIII kills HIV and hepatitis B virus, nearly eliminating the threat of infection. Consequently, AIDS mortality among those with hemophilia A has decreased markedly since 1995.
Identification and sequencing of the factor VIII gene has led to a number of insights. Patients with nonsense or frameshift mutations usually develop severe hemophilia, and those with missense mutations usually have mild to moderate disease. This is expected because nonsense and frameshift mutations typically produce a transcript or truncated protein that is degraded and lost. Missense mutations produce a single amino acid substitution without a dominant negative effect, usually resulting in an altered but partially functional protein product. Many of the point mutations take place at methylated CG sequences, which are hot spots for mutation (see Chapter 3 ). About 45% of severe cases of hemophilia A are caused by a chromosome inversion (see Chapter 6 ) that disrupts the factor VIII gene. An additional 5% of patients have deletions, which usually lead to relatively severe disease. About 10% of female heterozygotes have factor VIII levels less than 35%, and some of these are manifesting heterozygotes (see text), with mild symptoms of hemophilia A.
Cloning of the factor VIII gene has enabled the production of human factor VIII using recombinant DNA techniques. Extensive clinical testing showed that recombinant factor VIII works as effectively as the donor-derived form, and it was approved for commercial use in 1994. Recombinant factor VIII has the advantage that there is no possibility of viral contamination. However, as with other forms of factor VIII, recombinant factor VIII generates antifactor VIII antibody production in approximately 10% to 15% of patients. (This response is most common in patients who have no native factor VIII production.)
Two other major bleeding disorders are hemophilia B and von Willebrand disease. Hemophilia B, sometimes called Christmas disease, ∗
∗ Christmas was the last name of the first reported patient.
is also an X-linked recessive disorder and is caused by a deficiency of clotting factor IX. This condition is about one-fifth as common as hemophilia A and can be treated with donor-derived or recombinant factor IX. Von Willebrand disease is an autosomal dominant disorder that is highly variable in expression. Although it can affect as many as 1% of individuals of European descent, it reaches severe expression in fewer than 1 in 10,000. The von Willebrand factor, which is encoded by a gene on chromosome 12, acts as a carrier protein for factor VIII. In addition, it binds to platelets and to damaged blood vessel endothelium, thus promoting the adhesion of platelets to damaged vessel walls.Hemophilia is of historical interest because it affected members of the royal families of Germany, Spain, England, and Russia ( Fig. 5.4 ). Among these families, Queen Victoria of England was the first known heterozygous hemophilia carrier. She had one affected son, and two of her daughters had affected sons, making them presumptive carriers. One of her affected great-grandsons was the Tsarevitch Alexei of Russia, the son of Tsar Nicholas II and Alexandra. Grigori Rasputin, called the “mad monk,” had an unusual ability to calm the Tsarevitch during bleeding episodes, probably through hypnosis. As a result he came to have considerable sway in the royal court, and some historians believe that his destabilizing influence helped to bring about the 1917 Bolshevik revolution. Recently, the Russian royal family was again touched by genetics. Using the polymerase chain reaction, autosomal DNA microsatellites and mitochondrial DNA sequences were assayed in the remains of several bodies exhumed near Yekaterinburg, the reputed murder site of the royal family. Analysis of this genetic variation and comparison with living maternal relatives showed that the bodies were indeed those of the Russian royal family. Further analysis demonstrated that Alexei had a pathogenic mutation in the gene that encodes factor IX, establishing that the royal families were affected by hemophilia B (rather than the more common hemophilia A).

Muscular dystrophy, defined as a progressive weakness and loss of muscle, exists in dozens of different forms. Of these, Duchenne muscular dystrophy (DMD), named after the French neurologist who provided the first comprehensive description in 1868, is one of the most severe and common forms. It affects approximately 1 of every 4000 males, a prevalence figure that is similar among all ethnic groups studied thus far.
The symptoms of DMD are usually seen before the age of 5 years, when parents begin to notice unusual clumsiness and muscle weakness. Pseudohypertrophy of the calves, the result of infiltration of muscle by fat and connective tissue, is often seen early in the course of the disease. All skeletal muscle degenerates eventually, and most patients with DMD are confined to a wheelchair by 11 years of age. The heart and respiratory musculature become impaired, and death usually results from respiratory or cardiac failure. Survival beyond age 25 years is uncommon; little can be done to alter the ultimate course of this disease.
As muscle cells die, the enzyme creatine kinase (CK) leaks into the blood stream. In DMD patients, serum CK is elevated at least 20 times above the upper limit of the normal range. This elevation can be observed presymptomatically, before clinical symptoms such as muscle wasting are seen. Other traditional diagnostic tools include electromyography, which reveals reduced action potentials, and muscle biopsy.
Female heterozygous carriers of the DMD-causing mutations are usually free of disease, although 8% to 10% have some degree of muscle weakness. In addition, serum CK exceeds the 95th percentile in approximately two-thirds of heterozygotes.
Until the gene responsible for DMD was identified in 1986, little was known about the mechanism responsible for muscle deterioration. The DMD gene covers approximately 2.4 Mb of DNA, making it the largest gene known in the human. It contains 79 exons that produce a 14-kb mRNA transcript. Because of this gene’s huge size, transcription of an mRNA molecule can take as long as 24 hours. The protein product, named dystrophin, was unknown before the identification of DMD. Dystrophin accounts for only about 0.002% of a striated muscle cell’s protein mass and is localized on the cytoplasmic side of the cell membrane. Its amino terminus binds F-actin, a key cytoskeletal protein. The carboxyl terminus of dystrophin binds a complex of glycoproteins known as the dystroglycan–sarcoglycan complex, which spans the cell membrane and binds to extracellular proteins. Dystrophin thus links these two cellular components and plays a key role in maintaining the structural integrity of the muscle cell. Lacking dystrophin, the muscle cells of the DMD patient gradually die as they are stressed by muscle contractions.
The large size of DMD helps to explain its high mutation rate, about 10 –4 per locus per generation. As with the gene responsible for neurofibromatosis type 1, the DMD gene presents a large target for mutation. A slightly altered form of the DMD gene product is normally found in brain cells, in which the transcription initiation site is farther downstream in the gene and a different promoter is used. Thus the mRNA transcript and the resulting gene product differ from the gene product found in muscle cells. Dystrophin’s expression in the brain helps to explain why approximately 25% of DMD patients have some degree of intellectual disability. Several additional promoters have also been found, providing a good example of a single gene that can produce different gene products as a result of modified transcription.
Becker muscular dystrophy (BMD), another X-linked recessive dystrophic condition, is less severe than the Duchenne form. The progression is also much slower, with onset at 11 years of age, on average. One study showed that whereas 95% of DMD patients are confined to a wheelchair before 12 years of age, 95% of those with BMD become wheelchair bound after 12 years of age. Some never lose their ability to walk. BMD is less common than DMD, affecting about 1 in 18,000 male births.
For some time it was unclear whether BMD and DMD are caused by distinct X-linked loci or by different mutations at the same locus. Identification of DMD showed the latter to be the case. Both diseases usually result from deletions (65% of DMD cases and 85% of BMD cases) or duplications (6% to 7% of DMD and BMD cases) in DMD. Whereas the great majority of DMD-causing deletions and duplications produce frameshifts, the majority of BMD-causing mutations are in-frame alterations (i.e., a multiple of three bases is deleted or duplicated). One would expect that a frameshift, which is likely to produce a premature stop codon (see Chapter 3 ) and no protein product, would produce more severe disease than would an in-frame alteration.
The consequences of these different mutations can be observed in the gene product. Although dystrophin is absent in almost all DMD patients, it is usually present in reduced quantity (or as a shortened form of the protein) in BMD patients. Thus a dystrophin assay can help to distinguish between the two diseases. This assay also helps to distinguish both diseases from other forms of muscular dystrophy, because several of these forms (e.g., various limb–girdle muscular dystrophies) result from mutations in genes that encode proteins of the dystroglycan–sarcoglycan complex, whereas dystrophin appears to be affected only in BMD and DMD ( Figs. 5.5, 5.6, and 5.7 ).



Genetic testing (see Chapter 13 ) is now commonly used to help diagnose DMD and BMD; a disease-causing mutation is identified in about 95% of cases. Considerable research has been done on genetically based therapies for muscular dystrophy. For example, small-molecule drugs can cause ribosomes to read through premature stop codons and have shown promise in mouse models. Antisense oligonucleotides (see Chapter 13 ) can induce the skipping of the altered DMD exon during mRNA translation. These approaches, along with gene therapy in which a normal copy of DMD is inserted into muscle cells, are now being tested in clinical trials.
Human vision depends on a system of retinal photoreceptor cells, about 95% of which are rod cells. They contain the light-absorbing protein rhodopsin and allow us to see in conditions of dim light. In addition, the retina contains three classes of cone cells, which contain light-absorbing proteins (opsins) that react to light wavelengths corresponding to the three primary colors—red, green, and blue. Color vision depends on the presence of all four of these cell types. Because three major colors are involved, normal color vision is said to be trichromatic.
There are many recognized defects of human color vision. The most common of these involve red and green color perception and have been known since 1911 to be inherited in X-linked recessive fashion. Thus they are much more common in males than in females. Various forms of red–green colorblindness are seen in about 8% of European males, 4% to 5% of Asian males, and 1% to 4% of African and Native American males. Among European males, 2% are dichromatic: they are unable to perceive one of the primary colors, usually red or green. The inability to perceive green is termed deuteranopia, and the inability to perceive red is termed protanopia. About 6% of European males can detect green and red, but with altered perception of the relative shades of these colors. These are respectively termed deuteranomalous and protanomalous conditions.

It should be apparent that dichromats are not really color blind because they can still perceive a fairly large array of different colors. True colorblindness ( monochromacy, the ability to perceive only one color) is much less common, affecting approximately 1 in 100,000 persons. There are two major forms of monochromatic vision. Rod monochromacy is an autosomal recessive condition in which all visual function is carried out by rod cells. Blue cone monochromacy is an X-linked recessive condition in which both the red and green cone cells are absent.
Cloning of the genes responsible for color perception has revealed a number of interesting facts about both the biology and the evolution of color vision in humans. In the 1980s, Jeremy Nathans and colleagues reasoned that the opsins in all four types of photoreceptor cells might have similar amino acid sequences because they carry out similar functions. Therefore the DNA sequences of the genes encoding these proteins should also be similar. But none of these genes had been located, and the precise nature of the protein products was unknown. How could they locate these genes?
Fortunately the gene encoding rhodopsin in cattle had been cloned. Even though humans and cattle are separated by millions of years of evolution, their rhodopsin proteins still share about 40% of the same amino acid sequence. Thus the cattle (bovine) rhodopsin gene could be used as a probe to search for a similar DNA sequence in the human genome. A portion of the bovine rhodopsin gene was converted to single-strand form, radioactively labeled, and hybridized with human DNA (much in the same way that a probe is used in Southern blotting [see Chapter 3 ]). Low-stringency hybridization conditions were used; temperature and other conditions were manipulated so that complementary base pairing would occur despite some sequence differences between the two species. In this way the human rhodopsin gene was identified and mapped to chromosome 3.
The next step was to use the human rhodopsin gene as a probe to identify the cone-cell opsin genes. Each of the cone-cell opsin amino acid sequences shares 40% to 45% similarity to the human rhodopsin amino acid sequence. By probing with the rhodopsin gene, the gene for blue-sensitive opsin was identified and mapped to chromosome 7. This gene was expected to map to an autosome because variants in blue sensitivity are inherited in autosomal recessive fashion. The genes for the red- and green-sensitive opsins were also identified in this way, and as expected were found to be on the X chromosome. The red and green genes are highly similar, sharing 98% of their DNA sequence.
Initially many investigators expected that people with color vision defects would display the usual array of deletions and missense and nonsense mutations seen in other disorders. But further study revealed some surprises. It was found that the red and green opsin genes are located directly adjacent to each other on the distal long arm of the X chromosome and that normal persons have one copy of the red gene but can have one to several copies of the green gene. The multiple green genes are 99.9% identical in DNA sequence, and the presence of multiple copies of these genes has no effect on color perception because only the red gene and the first green gene are expressed in the retina. However, when there are no green genes, deuteranopia is produced. Persons who lack the single red gene have protanopia.
The unique aspect of these deletions is that they are the result of unequal crossover during meiosis. Unlike ordinary crossover, in which equal segments of chromosomes are exchanged (see Chapter 2 ), unequal crossover results in a loss of chromosome material on one chromosome homolog and a gain of material on the other. Unequal crossover seems to be facilitated by the high similarity in DNA sequence among the red and green genes: It is relatively easy for the cellular machinery to make a mistake in deciding where the crossover should occur. A female with one red gene and two green genes could produce one gamete containing a red gene with one green gene and another gamete containing a red gene with three green genes. Unequal crossover could also result in gametes with no copies of a gene, producing protanopia or deuteranopia.

Unequal crossover also explains protanomalous and deuteranomalous color vision. Here crossover takes place within the red or green genes, resulting in new chromosomes with hybrid genes (e.g., a portion of the red gene fused with a portion of the green gene). The relative ratio of red and green components of these fusion genes determines the extent and nature of the red-green anomaly.
Because the opsin genes have DNA sequence similarity and perform similar functions, they are members of a gene family, much like the globin genes (see Chapter 3 ). This suggests that they evolved from a single ancestral gene that, through time, duplicated and diverged to encode different but related proteins. Evidence for this process is provided by comparing these genes in humans and other species. Because the X-linked red and green opsin genes share the greatest degree of DNA sequence similarity, we would expect that these two genes would be the result of the most recent duplication. Indeed, humans share all four of their opsin genes with apes and Old World monkeys, but the less closely related New World monkeys have only a single opsin gene on their X chromosomes. It is therefore likely that the red-green duplication occurred sometime after the split of the New and Old World monkeys, which took place about 30 to 40 million years ago. Similar comparisons date the split of the X-linked and autosomal cone opsin genes to approximately 500 million years ago. And finally, comparisons with the fruit fly, Drosophila melanogaster, indicate that the duplication that produced the rod and cone visual pigment genes may have occurred as much as 1 billion years ago.
Name | Gene | Clinical Characteristics |
---|---|---|
Juvenile retinoschisis | RS1 | Progressive visual impairment caused by splitting of the nerve fiber layer of the retina; begins in the first or second decade of life; impairment typically 20/60 to 20/120 |
Leri–Weill dyschondrosteosis | SHOX | Madelung deformity of the radius and ulna; mesomelia (shortening of the forearms and lower legs); short stature |
ATR-X | ATRX | Mental retardation; genital anomalies; and α-thalassemia without abnormalities of the α-globin gene complex |
Hypohidrotic ectodermal hypoplasia | EDA | Diminished sweating and heat intolerance; sparse and light-colored hair, eyelashes, and eyebrows; abnormal and/or missing teeth; recurrent upper airway infections |
Vitamin D–resistant rickets | PHEX | Hypophosphatemia due to reduced renal phosphate reabsorption; short stature; bowed legs; poor teeth formation |
Aarskog–Scott syndrome (faciogenital dysplasia) | FGD1 | Short stature; hypertelorism; genital anomalies |
Cleft palate with ankyloglossia | TBX22 | Cleft palate with or without ankyloglossia (tongue tie) |
Pelizaeus–Merzbacher disease | PLP1 | Defect of myelination; typically manifests in infancy or early childhood; characterized by nystagmus, hypotonia, spasticity, early death |
Nephrogenic diabetes insipidus | AVPR2 | Impaired response to antidiuretic hormone that leads to inability to concentrate urine, polydipsia (excessive thirst), polyuria (excessive urine production) |
Otopalatodigital spectrum disorders | FLNA | Skeletal dysplasia ranging from mild to lethal; males more severely affected than females |
Because females inherit two copies of the X chromosome, they can be homozygous for a disease-causing allele at a given locus, heterozygous at the locus, or homozygous for the normal allele at the locus. In this way, X-linked loci in females are much like autosomal loci. However, for most X-linked loci, there is only one copy of the allele in an individual somatic cell because of X inactivation. This means that about half of the cells in a heterozygous female will express the disease allele and half will express the normal allele.
The heterozygote will produce about 50% of the normal level of the gene product, as with autosomal recessive traits. Ordinarily this is sufficient for a normal phenotype. The situation is different for males, who are hemizygous for the X chromosome. If a male inherits a recessive disease gene on the X chromosome, he will be affected by the disease because the Y chromosome does not carry a normal allele to compensate for the effects of the disease allele.
An X-linked recessive disease with gene frequency q will be seen in a fraction q of males. This is because a male, having only one X chromosome, will manifest the disease if his X chromosome contains the disease-causing mutation. Females, needing two copies of the mutant allele to express the disease, will have a disease frequency of only q 2 , as in autosomal recessive diseases. For example, hemophilia A (see Clinical Commentary 5.1 ) is seen in about 1 of every 10,000 males in some populations. In a collection of 10,000 male X chromosomes, 1 chromosome would, on average, contain the disease-causing mutation ( q = 0.0001). Affected female homozygotes are almost never seen, because q 2 = 0.00000001, or 1 in 100,000,000. This example shows that, in general, males are more frequently affected with X-linked recessive diseases than are females, with this difference becoming more pronounced as the disease becomes rarer.
Because females have two copies of the X chromosome and males have only one (hemizygosity), X-linked recessive diseases are much more common among males than among females.
In addition to their higher prevalence in males than females, X-linked recessive diseases display several patterns of inheritance that distinguish them from autosomal dominant and recessive diseases ( Fig. 5.8 ). Because a father can transmit only a Y chromosome to his son, X-linked genes are not passed from father to son. (In contrast, father-to-son transmission can be observed for autosomal disease alleles.) An X-linked disease allele can be transmitted through a series of phenotypically normal heterozygous females, causing the appearance of skipped generations. The gene is passed from an affected father to all of his daughters, who as carriers transmit it to approximately half of their sons, who are affected.
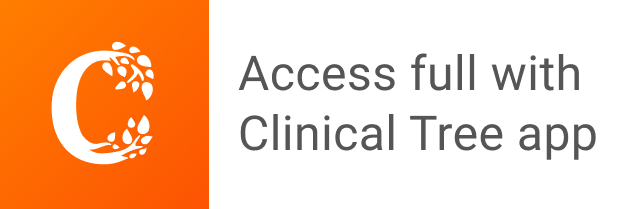