Developmental genetics is the study of how the instructions encoded in genes control and coordinate development of an organism, beginning with fertilization and ending with death. The field is important to health-care providers because mutations in genes can perturb developmental processes resulting in increased risk for birth defects, intellectual disability, and cancer. Worldwide, about 3% of all live-born children have a major birth defect (i.e., one that has a substantial impact on health), so ≈8 million children are born each year with a major birth defect. Birth defects are the leading cause of infant ∗
∗ An infant is a person who is younger than 1 year.
death in the United States and are associated with substantial morbidity.Birth defects can be isolated abnormalities, or they can be features of one of several thousand known genetic syndromes. The etiology of most birth defects is unknown; however, a substantial fraction is caused by mutations in genes that control normal development, either alone or in combination. The characterization of genes and developmental processes that coordinate animal development has revolutionized our understanding of the molecular basis of human birth defects. This chapter provides a brief review of the genes and proteins that control development and then discusses some of the cardinal developmental processes that, when disturbed, cause birth defects.
Development
Basic Concepts
Animal development can be defined as the process by which a fertilized ovum becomes a mature organism capable of reproduction. A single fertilized egg divides and grows to form different cell types, tissues, and organs, all of which are arranged in a species-specific body plan (i.e., the arrangement and pattern of body parts). Many of the instructions necessary for normal development are encoded by an animal’s genes. Because the genes in each cell of an organism are identical, several questions arise: How do cells with identical genetic constitutions form a complex adult organism composed of many different cells and tissues? What controls the fate of each cell, instructing a cell to become, for example, a brain cell or a liver cell? How do cells organize into discrete tissues? How is the body plan of an organism determined? Answering such fundamental questions has been a major focus of developmental biology for more than a century. The pace of discovery has accelerated dramatically in the past several decades, and these discoveries are helping us to understand the causes of human malformations and genetic syndromes.
For ethical and technical reasons, it is difficult to study early developmental events in human embryos. Consequently, a variety of nonhuman model organisms are used to facilitate the study of development ( Table 10.1 ). This approach is feasible because the major elements (genes and pathways) that control animal development are conserved across a wide range of species and body plans. In addition, many regulatory switches and signaling pathways are used repeatedly during development to control various patterning and differentiation events. This underscores the point that the evolution of species proceeds, in part, by continual tinkering with similar developmental programs to effect changes in an organism’s phenotype.
Organism | Generation Time ∗ | Advantages | Disadvantages |
---|---|---|---|
Caenorhabditis elegans (roundworm) | 9 days | Fate of every cell known Genome well characterized | Alternative body plan compared to vertebrates |
Easy to breed and maintain | Tissues cannot be cultured | ||
Drosophila melanogaster (fruit fly) | 10 days | Easy to breed Large populations Vast database of mutants Feasible and affordable to do large screens | Alternative body plan compared to vertebrates Must be stored live; cannot be frozen Pathology often different compared to humans |
Danio rerio (zebrafish) | 3 months | Transparent embryo Easy to maintain Large populations Feasible and affordable to do large screens | Targeted gene modification difficult |
Xenopus laevis (frog) | 12 months | Transparent embryo is large and easy to manipulate | Tetraploid genome makes genetic experiments difficult |
Gallus gallus (chicken) | 5 months | Easy to observe and manipulate embryo | Genetic experiments difficult |
Mus musculus (mouse) | 2 months | Pathology similar to humans Excellent tools for phenotypic characterization Targeted gene modification straightforward Fully annotated genome available | Relatively expensive to maintain Manipulation of embryo is challenging |
Papio hamadryas (baboon) | 60 months | Pathology and physiology similar to that of humans | Very expensive to maintain Small populations Long generation time Strong ethical concerns with use of primates |
∗ Generation time is defined as the age at which the organism is first capable of reproduction.
For example, ectopic expression (i.e., expression of the gene product in an abnormal location) of the Drosophila gene eyeless results in the formation of a well-formed but misplaced eye ( Fig. 10.1 ). Mice have a homologous gene, Pax6, †
† It is conventional to capitalize all letters of the names of human genes but only the first letter of the names of mouse genes, except for recessive mutations, which begin with a lower-case letter.
in which mutations can produce abnormally small eyes. When inserted ectopically into Drosophila, Pax6 again produces a misplaced fly eye. Mutations in the human homolog, PAX6, cause defects of the eye such as cataracts and aniridia (absence of the iris). PAX6, Pax6, and eyeless are homologous genes that encode DNA transcription factors (see Chapter 2 ). Although the ancestors of Drosophila and mouse diverged from the lineage leading to humans 500 million and 60 million years ago, respectively, the genes and pathways involved in development of the eye have been conserved.Approximately 2% to 3% of babies are born with a recognizable birth defect. Many birth defects are caused by mutations in genes encoding elements in pathways that control development. These pathways are highly conserved among animal species. Thus studies of nonhuman animal models are invaluable for understanding human development and the causes of birth defects.

A Brief Overview of Major Processes in Embryonic Development
Several major processes are involved in the development of the embryo. These include axis specification, pattern formation, and organogenesis. As the name suggests, pattern formation describes a series of steps in which differentiated cells are arranged spatially to form tissues and organs. The interactions of these cells are mediated by processes such as induction, which occurs when the cells of one embryonic region influence the organization and differentiation of cells in a second region. Axis specification involves the definition of the major axes of the body: ventral/dorsal, anterior/posterior, medial/lateral, and left/right. Specification of polarity (direction) is an important part of this process. As the axes are specified, the formation of organs and limbs (organogenesis) begins. Each of these major processes involves many different proteins that form structures and provide signals to coordinate the development of the embryo. The major types of these proteins, and the genes that encode them, are described next.
Embryonic development involves the processes of pattern formation, axis specification, and organogenesis. Each of these processes is controlled by a series of proteins that provide signals and form structures necessary for normal development of the embryo.
Genetic Mediators of Development: The Molecular Toolbox
The genes required for normal development encode many different products including signaling molecules and their receptors, DNA transcription factors, components of the extracellular matrix, enzymes, transport systems, and other proteins. Each of these genetic mediators is expressed in combinations of spatially and temporally overlapping patterns that control different developmental processes. As detailed in this chapter, mutations in the genes mediating development are a common cause of human birth defects.
Paracrine Signaling Molecules
Interactions between neighboring cells are usually mediated by proteins that can diffuse across small distances to induce a response. These molecules are often called paracrine factors because they are secreted into the space surrounding a cell (unlike hormones, which are secreted into the blood stream). Closely related paracrine factors have been isolated from a variety of organisms, making it clear that homologous molecules are used throughout the animal kingdom. To date, four major families of paracrine signaling molecules have been described: the Fibroblast Growth Factor (FGF) family, the Hedgehog family, the Wingless (Wnt) family, and the Transforming Growth Factor β (TGF-β) family. Each of these signaling molecules binds to one or more receptors to effect a response, and mutations in genes encoding these molecules may lead to abnormal communication between cells. Clinical Commentary 10.1 discusses the FGF family and associated receptors; the other three families are described in this section.
The four fibroblast growth factor receptors (FGFRs) are highly homologous glycoproteins, with a common structure that consists of a signal peptide (an amino acid sequence that helps to direct the protein to its proper cellular location), three immunoglobulin-like (Ig-like) domains, a membrane-spanning segment, and an intracellular tyrosine kinase domain. FGFRs are receptors for 22 fibroblast growth factors (FGFs), which participate in a wide variety of biological processes that include cell migration, growth, and differentiation (e.g., limb formation, brain patterning, etc). With varying affinities, FGFs bind FGFRs, which leads to phosphorylation and hence activation of the tyrosine kinase domain.
FGFRs are widely expressed in developing bone, and many common human autosomal dominant disorders of generalized bone growth (i.e., skeletal dysplasias) are caused by mutations in FGFR genes. The most prevalent of these disorders, affecting more than 250,000 people worldwide, is achondroplasia (ACH), which is characterized by disproportionate short stature (i.e., the limbs are disproportionately shorter than the trunk) and macrocephaly (see Chapter 4 ). Nearly all persons with ACH have a glycine → arginine substitution in the transmembrane domain of FGFR3, resulting in constitutive FGFR3 activation ( Fig. 10.3 ).

FGFR3 is normally expressed in resting chondrocytes where it restrains chondrocyte proliferation and differentiation. Overactivation causes further inhibition of chondrocyte growth, which produces skeletal defects. The degree of FGFR3 activation, which varies depending on the domain that is altered, corresponds to the severity of long-bone shortening. Lesser degrees of activation result in the milder skeletal abnormalities observed in hypochondroplasia, whereas markedly increased activation causes a virtually lethal short-stature syndrome called thanatophoric dysplasia.
These findings suggest that a potential treatment strategy might be to reduce or block the activity of FGFR3 or antagonize its downstream effect. Such therapeutic agents currently in testing include antibodies to the FGFR3 protein, decoy FGFR3, and BN111, an analog of C-natriuretic peptide. BN111 downregulates the protein kinase pathway activated by FGFR3, and early clinical trials have shown that it significantly increases linear growth in children with achondroplasia. In mice, FGFR3 -inactivating mutations cause expansion of the zones of proliferating cartilage and increased long bone growth, resulting in mice that are longer than average. A similar average increase in height has been observed in humans with a rare syndrome called CATSHL syndrome that is caused by a FGFR3 mutation predicted to reduce FGFR3 function.
Abnormal bone growth is also a feature of a group of autosomal dominant disorders characterized by premature fusion (synostosis) of the cranial sutures, misshapen skulls, and various types of limb defects. Collectively, these disorders are called craniosynostosis syndromes. Mutations in FGFR1, FGFR2, and FGFR3 cause at least ten distinct craniosynostosis disorders, the best-known of which is Apert syndrome. The same mutation can sometimes cause two or more different craniosynostosis syndromes. For example, a cysteine → tyrosine substitution in FGFR2 can cause either Pfeiffer or Crouzon syndrome. This suggests that additional factors, such as modifying genes, are partly responsible for creating different phenotypes ( Fig. 10.4 ) ( Table 10.2 ).

Gene | Syndrome | Characteristic |
---|---|---|
FGFR1 | Pfeiffer | Broad first digits, hypertelorism |
FGFR2 | Antley-Bixler | Mid-face hypoplasia, humeroradial synostosis |
Apert | Mid-face hypoplasia, fusion of digits | |
Pfeiffer | Broad first digits, hypertelorism | |
Crouzon | Mid-face hypoplasia, ocular proptosis | |
Beare–Stevenson | Mid-face hypoplasia, corrugated skin | |
Jackson–Weiss | Mid-face hypoplasia, foot anomalies | |
FGFR3 | Crouzon | Mid-face hypoplasia, ocular proptosis, acanthosis nigricans ∗ |
Muenke (nonsyndromic craniosynostosis) | Mid-face hypoplasia, brachydactyly, hearing loss |
∗ Acanthosis nigricans is characterized by hyperplastic and hypertrophic skin of varying pigmentation, most often covering the axillae, neck, genitalia, and flexural surfaces.
Name of Condition | Clinical Characteristics | Causal Genes(s) |
---|---|---|
Primary ciliary dyskinesia | Chronic airway disease, laterality defects | >30 genes |
Polycystic kidney disease (AR) | Early onset kidney and liver cysts, hypertension, renal failure | PKHD1 |
Polycystic kidney disease (AD) | Late onset kidney cysts, vascular aneurysms | PKD1 , PKD2 , GANAB , or DNAJB11 |
Bardet-Biedl syndrome | Rod-cone dystrophy, obesity, polydactyly, hypogonadism | >19 genes |
Joubert syndrome | Developmental delay, hypotonia, cerebellar malformation | >34 genes |
Meckel-Gruber syndrome | Occipital encephalocele, cystic kidneys, fibrotic liver, polydactyly | >14 genes |
Short-rib polydactyly | Constricted thoracic cage, short ribs, shortened tubular bones | >18 genes |
Oral-facial-digital syndromes | Facial, brain, kidney abnormalities | >15 genes |
Nephronophthisis | Reduced renal concentrating ability, chronic tubulointerstitial nephritis, fibrocystic renal disease | >17 genes |
The first member of the Hedgehog family was originally isolated in a Drosophila mutant that has bristles in an area that is naked in the normal fly (hence the name “hedgehog”). Vertebrates have several homologs of hedgehog, of which the most widely used of is termed Sonic hedgehog (Shh). Among its many roles Shh participates in axis specification, induction of motor neurons within the neural plate, and patterning of the limbs. The primary receptor of Shh is a transmembrane protein encoded by a gene called patched. The normal action of patched is to inhibit the function of another transmembrane protein called smoothened, encoded by the gene Smo . Binding of Shh to the patched receptor results in disinhibition of smoothened and activation of an intracellular signaling cascade that targets members of the GLI family of transcription factors ( Fig. 10.2 ).

Mutations in the human homolog, PATCHED (PTC), cause Gorlin syndrome (see Fig. 10.2 ), a disorder characterized by rib anomalies, cysts of the jaw, and basal cell carcinomas (a form of skin cancer). Somatic mutations in PTC have also been found in sporadic basal cell carcinomas. Germline mutations in PTC alter the regulation of developing cells to cause birth defects, and somatic mutations in PTC can alter the regulation of terminally differentiated cells to cause cancer.
The Wnt family of genes is named after the Drosophila gene wingless and one of its vertebrate homologs, integrated. The wingless gene establishes polarity during Drosophila limb formation, and members of the Wnt family play similar roles in vertebrates. Wnt genes encode secreted glycoproteins that bind to members of the frizzled and low-density lipoprotein (LDL) receptor–related protein families. In humans, 19 different Wnt genes have been identified, and they participate in multiple signaling pathways including the canonical Wnt (also known as the Wnt-β-catenin) pathway and the two noncanonical (i.e., independent of β-catenin) planar cell polarity and Wnt/calcium pathways. These pathways regulate a wide variety of developmental processes, including specification of the dorsal/ventral axis and formation of the brain, muscle, gonads, and kidneys. Pathogenic variants in WNT genes cause a variety of genetic conditions in humans, including tetra-amelia or absence of all four limbs (WNT3), Robinow syndrome (WNT5A), deficiency of the ulna and fibula (WNT7A), and odontoonychodermal dysplasia (WNT10A). Abnormal Wnt signaling has also been associated with the formation of tumors.
The TGF-β supergene family ‡
‡ A supergene family is a group of related gene families.
is composed of a large group of structurally related genes that encode proteins that form homodimers or heterodimers. Members of the TGF-β supergene family include the TGF-β family itself, the bone morphogenetic protein (BMP) family, the activin family, and the Vg1 family. Although the role of BMPs is not limited to bone development, members of the BMP family were originally isolated because of their ability to induce bone formation.Mutations in a member of the BMP family, cartilage-derived morphogenetic protein 1 ( CDMP 1), cause various skeletal abnormalities. Different mutations can produce distinct phenotypes (allelic heterogeneity; see Chapter 4 ). For example, a nonsense mutation in CDMP1 causes dominantly inherited brachydactyly (short digits). Persons homozygous for a 22-bp duplication in CDMP1 have brachydactyly as well as shortening of the long bones of the limbs in an autosomal recessive disorder called acromesomelic dysplasia. A homozygous missense mutation produces autosomal recessive Grebe chondrodysplasia, also characterized by severe shortening of the long bones and digits. The mutant protein is not secreted, and it is thought to inactivate other BMPs by forming heterodimers with them and preventing their secretion. Thus mutations causing Grebe chondrodysplasia produce a novel type of dominant negative effect by inactivating the products of other genes.
To effect a response, extracellular signals must be transduced by a cell. One of the best known of these signal-transduction systems is the RTK/Ras GTPase/MAPK §
§ GTPase, guanosine triphosphatase; MAPK, mitogen-activated protein kinase; RTK, receptor tyrosine kinase.
(RTK-MAPK) signaling pathway. The RTK-MAPK pathway regulates various cellular functions such as gene expression, division, differentiation, and death. Accordingly, the RTK-MAPK pathway is used widely during development. Mutations in genes that encode several components of the RTK-MAPK pathway have been found to cause human malformation syndromes ( Fig. 10.5 ). The best-known of these, Noonan syndrome, is characterized by short stature, characteristic facial features, webbing of the neck, and congenital heart disease—most commonly stenosis of the pulmonary outflow tract. About half of all cases of Noonan syndrome are caused by gain-of-function mutations in the protein tyrosine phosphatase, nonreceptor-type, 11 ( PTPN 11) gene, whereas mutations in each of at least seven other genes ( SOS1 , NRAS , KRAS , RIT1 , SHOC2 , RAF1 , and CBL ) explain most other cases. Each of these genes encodes a protein that is part of the RTK-MAPK pathway (see Fig. 10.5 ). The clinical characteristics of Noonan syndrome overlap with those of two rarer conditions, Costello syndrome and cardiofaciocutaneous syndrome (CFC) syndrome. These conditions are caused by mutations in unique and overlapping components of the RTK-MAPK pathway (see Fig. 10.5 ). Thus disruption of different components of the same signaling or developmental pathway cause different malformation syndromes with overlapping clinical features.
Other secreted proteins inhibit the function of BMPs. In humans, mutations in the gene Noggin, which encodes one of these inhibitors, cause fusion of the bones in various joints. In some affected persons, the joints initially appear to be normal. However, they are progressively obliterated by formation of excess cartilage that ultimately fuses the bones of the joint together (i.e., a synostosis) as the person ages. The primary joints affected are those of the spine, the middle ear bones, and the limbs, particularly the hands and feet. Affected persons experience progressively limited movement of these joints and develop hearing loss.
Paracrine signaling molecules are secreted, diffuse a short distance, and bind to a receptor that effects a response. There are four major families of paracrine signaling molecules: the fibroblast growth factor (FGF) family, the Hedgehog family, the Wingless (Wnt) family, and the Transforming Growth Factor β (TGF-β) family.
DNA Transcription Factors
There are many different ways to regulate the expression of a gene. For example, a gene might not be transcribed, the rate of transcription may be altered, or the transcribed mRNA might not be translated into protein. As discussed in Chapter 2 , genes encoding proteins that turn on (activate) or turn off (repress) other genes are called transcription factors. Transcription factors commonly do not activate or repress only a single target. Often they regulate the transcription of many genes, which in turn regulate other genes in a cascading effect. Consequently, mutations in transcription factor genes typically have pleiotropic effects.
There are many different families of transcription factors, members of which commonly share specific properties such as a common DNA-binding domain. Members of these different families have pivotal roles in controlling development, and alterations can cause birth defects. Examples include homeobox-containing genes such as the HOX, PAX, EMX, and MSX families; high-mobility group (HMG)-box–containing genes such as the SOX family; and the T-box family.
The HMG domain of SOX proteins appears to activate transcription indirectly by bending DNA so that other factors can make contact with promoter regions of genes. Several SOX genes act in different developmental pathways. The prototypic SOX gene is the SRY (sex-determining region of the Y chromosome) gene, which encodes the mammalian testis-determining factor (see Clinical Commentary 6.2 in Chapter 6 ). Sox 9 is expressed in the genital ridges of both sexes, but it is up-regulated in males and down-regulated in females before differentiation of the gonads. Sox 9 also regulates chondrogenesis and the expression of Col2A1, a collagen gene (see Chapter 2 ). As might be predicted from these expression and interaction patterns, mutations in SOX 9 cause a disorder characterized by skeletal defects (campomelic dysplasia) and sex reversal that produces XY females. Mutations in a related gene, SOX 10, result in a syndrome characterized by Hirschsprung disease (hypomotility of the bowel caused by a reduced number of enteric nerve cells), pigmentary disturbances, and deafness ( Clinical Commentary 10.2 ).
There are many different families of transcription factors, each of which regulates the transcription of specific genes. The same transcription factor is often used in different developmental pathways. Thus disorders caused by mutations in genes encoding transcription factors are often pleiotropic.
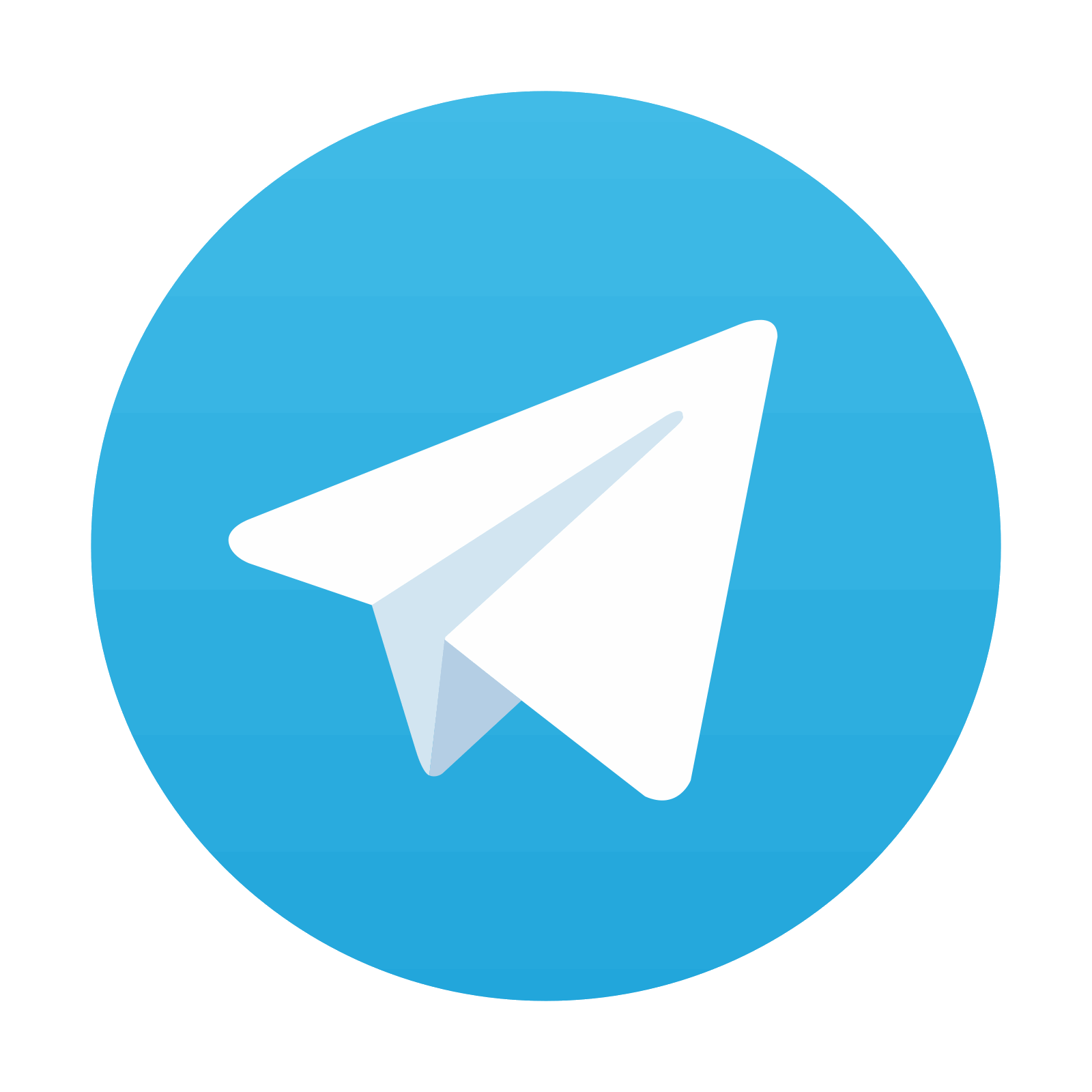
Stay updated, free articles. Join our Telegram channel
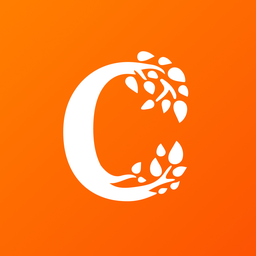
Full access? Get Clinical Tree
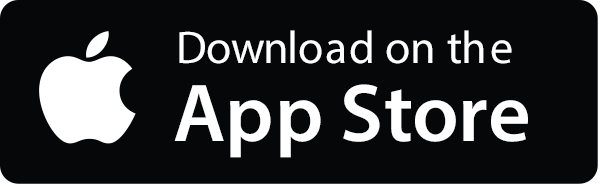
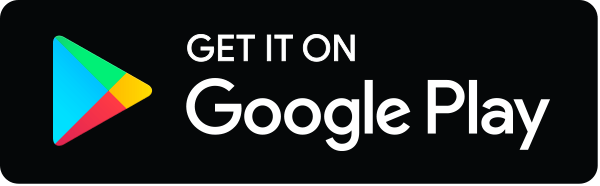
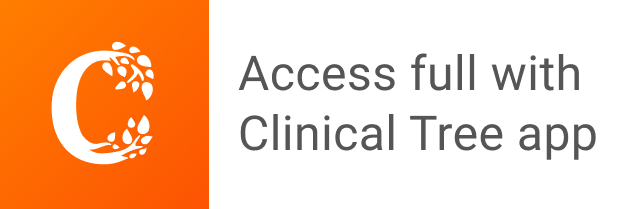