http://evolve.elsevier.com/Edmunds/NP/
A drug is any substance that is used in the diagnosis, cure, treatment, or prevention of a disease or condition (McKenry et al, 2005). This is a general definition that is not very useful because of the great diversity of characteristics and actions of drugs. To be more precise, pharmacokinetics is the study of the action of drugs in the body, including the processes of absorption, distribution, metabolism, and elimination. It may be thought of as what the body does to the drug. Pharmacodynamics is the study of the biochemical and physiologic effects of drugs on the function of living organisms and of their component parts (Brunton, Chabner, & Knollman, 2010). It includes consideration of the mechanisms of drug action and may be viewed as what the drug does to the body.
Note: These concepts are essential to an understanding of the prescribing role. Although many readers of this text may have had extensive experience in administering drugs, mastery of the content in this chapter is mandatory before moving to the more advanced role of drug prescriber. This chapter therefore is one of the most important in the book and presumes an extensive understanding of the scientific principles of biochemistry, anatomy, physiology, and pathophysiology. The information in this chapter is necessary for the provider to progress from memorizing drug names (an increasingly difficult task) to understanding the process of drug utilization and being able to generalize from basic principles. This chapter should be read more than once to best understand how to apply the principles to specific drugs. Not everything can be absorbed in one sitting.
A good understanding of physiology is necessary before one can understand the mechanisms of drug actions. The reader may find it useful to review relevant physiology before undertaking the study of a class of drugs. This chapter attempts to express clearly and simply some of the more complex principles of pharmacokinetics. Definitions or concepts that are important in the understanding of pharmacotherapeutics are emphasized. It is written at a deceptively simple level to help clinicians clearly understand major concepts that are commonly overlooked or those that are skimmed over quickly but never really mastered.
Drug Nomenclature
Drugs have three names: a chemical name, a generic name, and a trade, or brand, name. The chemical name describes the drug’s chemical composition and molecular structure. The generic name, or nonproprietary name, is the official name assigned by the manufacturer with the approval of the U.S. Adopted Name Council (USAN) and is the name listed in pharmacology reference books (McKenry et al, 2006). The trade name is the patent name given to the medicine by the company that is marketing the drug. If more than one company manufactures the drug, then the drug will have more than one trade name, thus adding to the confusion. Trade names are usually simpler and easier to remember than generic names because drug companies want you to remember their name and to use their drug. The generic name often will identify the type of drug that it is and thus will reveal the therapeutic effect. For example, it is important to identify a β-blocker by the similar-sounding generic names, such as atenolol, metoprolol, or propranolol, vs. learning the trade names (Tenormin, Lopressor, and Inderal, respectively). Providers should refer to drugs by their generic names when communicating with patients and other health care providers. This is very important with OTC medications because the contents of trade name products may vary.
Pharmacokinetics
Pharmacokinetics focuses on the processes concerned with absorption, distribution, biotransformation (metabolism), and excretion (elimination) of drugs (Figure 3-1).
Absorption
Absorption describes how the drug leaves its site of administration. The bioavailability of the product is what is most important clinically. Bioavailability is how much of the drug that is administered reaches its site of action (Brunton et al, 2010). The fraction of the drug that reaches the systemic circulation is called the f value. After a solid or liquid drug has been orally ingested, the drug must break up (disintegrate) and then become soluble in body fluids (dissolution) before the process of pharmacokinetic absorption begins (Stringer, 2005).
Bioequivalence is an issue with generic vs. trade drugs. Bioequivalence means that two drug products (1) contain the same active ingredients; (2) are identical in strength or concentration, dosage form, and route of administration; and (3) have essentially the same rate and extent of bioavailability (Brunton et al, 2010). Although a generic drug may have the same amount of drug, because of variability in the way the medication is manufactured, slightly more or less drug may be bioavailable. Legally, the drug must be ±20% of the proprietary drug. This variance becomes a concern with certain drugs, especially when the therapeutic window is narrow (e.g., Lanoxin vs. digoxin). Avoiding variation and complying with preferred drug formularies are the major reasons for ordering “Dispense as written” trade name prescriptions.
Factors That Affect Absorption
Four primary factors must be considered in evaluating drug absorption: drug characteristics, routes of administration, blood flow, and cell membrane characteristics.
Drug Characteristics: The following list includes some general drug characteristics relevant to all routes of administration. (Other factors are discussed under the specific route of administration.)
Routes of Administration: The complexity of routes of administration and delivery systems has increased greatly. The most common routes for giving medications include oral (PO), topical (TOP, TD), subcutaneous (SubQ), intramuscular (IM), intravenous (IV), and rectal (PR). Other less commonly used routes include intradermal (ID), sublingual (SL), buccal, intraarticular, inhalation, intravaginal, ophthalmic, and aural.
Oral: Oral ingestion is the most common method of drug administration. It is the most convenient and the most economical route; it is also the safest. However, disadvantages of oral administration include the following:
Many variations are available in oral drug formulations; in order from the fastest absorption rate to the slowest absorption rate, they include liquids, elixirs, syrups, suspensions, solutions, powders, capsules, tablets, coated tablets, enteric-coated tablets, and slow-release formulations (Brunton et al, 2010; Katzung et al, 2011).
Controlled-release preparations are designed to provide slow, uniform absorption of a drug (usually with a short half-life) over a long time, usually from 8 to 12 hours. These work with varying degrees of success. Some formulations are provided in a wax matrix that is not absorbed but is excreted in the feces (Brunton et al, 2010). Patients may become concerned about the appearance of this substance in the feces unless the clinician mentions this.
Sublingual Preparations: Some drugs that are nonionic and have high lipid solubility are readily absorbed by the oral mucosa. When drugs are absorbed orally, they must pass through the liver before they are distributed to the rest of the body. Many drugs are extensively metabolized on this first pass, allowing little of the drug to remain active. Drugs absorbed sublingually are advantageous because they avoid the first-pass phenomenon; therefore, the drug will reach the site of action quickly. Sublingual nitroglycerin is an example of a drug that needs to work quickly (chest pain).
Topical: Few drugs easily penetrate intact skin. Lipid-soluble drugs may not be absorbed because the skin acts as a lipid barrier. However, other types of solutions may be absorbed. Skin that is not intact will absorb drugs more readily. Thus, it is important to apply topical medications to intact, healthy skin to ensure the correct absorption. Mucous membranes also readily absorb drugs more readily than intact skin because of increased vascularity. Topical administration has the same advantage of avoiding the first-pass metabolism of the drug through the liver (Brunton et al, 2010).
Rectal: Medications that cannot be given orally often can be given rectally. Rectal medications usually are given when the patient is vomiting or unconscious. One advantage is that less first-pass metabolism of the drug through the liver occurs for rectal than for oral preparations. However, rectal absorption tends to be more inconsistent and less complete than oral absorption, and some drugs may cause rectal irritation. It is not always necessary to have a specially formulated rectal preparation (Edmunds, 2013). For example, oral timed-release morphine tablets will be absorbed rectally and are useful when the patient is unable to take an occasional oral dose of pain medicine.
Inhalation: Drugs can be given by nasal spray for local topical absorption through mucous membranes or by inhaler or nebulizer for pulmonary absorption. Pulmonary absorption uses a large surface area, making absorption rapid. Inhalation delivers the medication directly to the desired site of action, forcing particles of drugs down into the pulmonary system. This also avoids first-pass metabolism in the liver. The disadvantages of inhalation therapy include problems associated with regulating the exact dosage and the fact that many patients experience difficulty in self-administering a drug via inhaler.
Ophthalmic: Drops or ointments may be prescribed for the eye. These should be instilled into the pouch of the lower eyelid and should not be applied directly to the eye surface itself. Anything formulated for use on the eye can be used anywhere else in the body (Edmunds, 2013).
Blood Flow: Circulation at the site of administration is important in the drug absorption process. Decreased circulation (as seen in congestive heart failure) will result in decreased drug absorption (Katzung et al, 2011). For example, insulin injected into a thigh muscle, followed by exercise, will produce more rapid absorption of the insulin than occurs without exercise.
Cell Membrane Characteristics: When drugs are absorbed, they pass through cells, not between them; therefore, the drug must pass through the cellular wall or membrane. The structure of the cell membrane influences this process (Figure 3-2). The cell membrane is composed of a two-molecule layer of lipids that contains protein molecules between the lipids. It also contains carbohydrate molecules that are attached to the outer surface of the membrane. The proteins can be integral (in which case they go through the membrane) or peripheral (in which case they are attached to the surface of the membrane) (McCance & Huether, 2009). Integral proteins act as structural channels for the transportation of water-soluble substances (ions) or as carrier proteins in active transport. Peripheral proteins are enzymes. Glycoproteins may be antigenic sites in immune reactions or drug receptors. The pores permit the passage of small water-soluble substances such as water, electrolytes, urea, and alcohol (Brunton et al, 2010).
FIGURE 3-2 Cell membrane structure.
The lipid bilayer provides the basic structure and serves as a relatively impermeable barrier to most water-soluble molecules. (Modified from Thibodeau GA, Patton KI: Structure and function of the human body, ed 13, St Louis, 2008, Mosby.)
Drugs cross membranes via passive diffusion or active transport. Passive diffusion involves the random movement of drug molecules from high to low concentrations. Active transport moves molecules that are moderately sized, water soluble, or ionic across cell membranes. In active transport, these molecules form complexes with carriers for transport through the membrane and then dissociate from them. Active transport requires expenditure of energy and can occur against the concentration gradient (Stringer, 2005) (Figure 3-3).
FIGURE 3-3 Active mediated transport.
Metabolic energy is necessary for the active transport of many substances, including Na. (Modified from Alberts B et al, editors: Molecular biology of the cell, ed 3, New York, 1994, Garland.)
In passive diffusion, the drug molecule penetrates along a concentration gradient as a result of its solubility in the lipid layer of the membrane (Figure 3-4). This transfer occurs in proportion to the magnitude of the concentration gradient across the membrane. The higher the concentration, the more rapid the diffusion across the membrane. If the drug is not an electrolyte, a steady state is attained when the concentration of free drug is the same on both sides of the membrane. If the drug is an ionic compound, the steady-state concentration will depend on the difference in pH across the membrane (Brunton et al, 2010).
FIGURE 3-4 Passive diffusion.
Oxygen, nitrogen, water, urea, glycerol, and carbon dioxide can diffuse readily down the concentration gradient. Macromolecules are too large to diffuse through pores in the plasma membrane. Ions may be repelled if the pores contain substances with identical charges. (From Thibodeau GA, Patton KI: Understanding pathophysiology, ed 6, St Louis, 2008, Mosby.)
Whether the drug is lipophilic or hydrophilic affects absorption across cell membranes. Lipids pass through membranes better than hydrophilic molecules do. However, most cell membranes are permeable to water by diffusion or by hydrostatic or osmotic differences across the membrane (Stringer, 2005). The water may carry with it small water-soluble substances such as urea.
The pH of a drug also affects diffusion. Nonionized drugs are more lipid soluble and may readily diffuse across cell membranes. Ionized drugs are lipid insoluble and nondiffusible. Acidic drugs such as aspirin become nonionized in the acidic environment of the stomach and thus can diffuse across the membranes (DiPiro et al, 2011; Stringer, 2005). A change in the acidity of the stomach, as occurs with antacids, will affect absorption of drugs. Basic drugs such as alkaloids ionize in the stomach and are not well absorbed. These drugs are better absorbed in a less acidic environment such as the small intestine.
Active transport is a process used in the cell in three situations: when a particle is going from low to high concentration, when particles need help entering the membrane because they are selectively impermeable, and when very large particles enter and exit the cell. In order for active transport to occur, different types of pumps assist in the process:
Distribution
Distribution is the transport of a drug in body fluids from the bloodstream (at the site of absorption) to various tissues in the body. The pattern of distribution depends on the pharmacokinetic activity of different types of tissue and the different physicochemical properties of drugs (Brunton et al, 2010). Drugs vary in their ability to move into various body compartments (e.g., brain, fat, lung, eye). The best way to know how much of a given drug gets into a particular body compartment is to consult standard reference texts. Because blood is an easily accessible body fluid, blood concentrations often are studied to determine how they relate to drug concentrations in other body compartments. The dose-related effects of a drug are then correlated with a given blood concentration or range of concentration. Once a relationship has been established, blood concentrations can be used to monitor therapy.
Distribution consists of two phases. The first involves movement from the site of administration into the bloodstream. Delivery of the drug into the tissue at the site of action is the second phase of distribution (Katzung et al, 2011).
The volume of distribution (Vd) is a concept that is useful when one seeks to understand where the drug goes once it is absorbed. Volume of distribution is a description of the amount of space into which a drug can be spread or distributed. It is the calculated volume or size of a compartment necessary to account for the total amount of drug in the body if it were present throughout the body at the same concentration found in the plasma (Brunton et al, 2010). Because most drugs are not equally distributed, this is a theoretical concept and not a real volume. It is, however, useful in predicting drug concentrations, understanding how well a drug is absorbed into tissues, and understanding whether it will be accumulated in the tissues. If a drug has a small volume of distribution, it stays in the central compartment and is not widely distributed. If the drug has a large volume of distribution, it is found widely throughout the body. The larger the Vd, the more drug there is in the tissue. Because we are able to measure the amount of drug in the bloodstream only through serum levels, calculation of the volume of distribution allows us to estimate or predict the concentration of drug in the tissue (Stringer, 2005). The Vd may be calculated by examining the following relationships:
then
This means that the Vd is equal to the dose of drug in the body divided by the concentration in the blood (Brunton et al, 2010).
Water-soluble drugs have a Vd that is similar to the plasma volume because they are distributed into the blood. The plasma volume for a normal adult is about 3 to 5 liters. Lipophilic drugs have a larger Vd. The total fluid volume in the body is about 40 liters for a 70-kg (150-lb) person. Because the Vd of a lipophilic drug can exceed this volume, it is important to remember that this calculation is of a hypothetical volume, not a real volume. It is the volume that would be required to contain the entire drug in the body if the drug were distributed in the same concentration as in the blood or plasma (Brunton et al, 2010).
Factors that affect the volume of distribution include plasma protein binding, obesity, edema, and tissue binding. Therefore, the Vd for a given drug can change as a function of the patient’s age, gender, disease, and body composition.
Geriatric patients often have relatively less muscle and more fat, placing them at risk for accumulation of lipophilic drugs in the adipose tissue. If these patients lose weight and take the same dose, they could become toxic. This can be a particular problem with lipophilic benzodiazepines. In these patients, water-soluble drugs have a smaller Vd, resulting in increased blood concentrations. This effect is even greater if the patient is dehydrated. An example of a drug that may cause this problem is gentamicin. In addition, excess fluid in the interstitial spaces, such as is seen with edema, will affect the distribution of water-soluble drugs.
Any alteration in the normal muscle-to-fat ratio will change the Vd of a drug. In obesity, lipophilic drugs are distributed into adipose tissue and tend to accumulate. Thus, little drug is available elsewhere to produce an effect. For example, phenobarbital is fat soluble. The drug may become trapped in the fatty tissue, causing low blood levels of phenobarbital. Fat-soluble drugs are slowly released from the fat into the bloodstream, so they have a longer duration of action. This factor also may prolong the duration of side effects or may affect dosing schedules.
Drugs that are highly protein bound (>90%) have a volume of distribution that is about the same as the amount of plasma. This is a small volume of distribution. An example would be the thyroid hormones. If the patient has a decreased serum albumin, more active drug is available for protein-bound drugs. If two protein-bound drugs are used, the most tightly protein-bound drug will tend to displace the other. Examples of these drugs include furosemide and the cephalosporins (Brunton et al, 2010; DiPiro et al, 2011).
Another common change that may affect the distribution of drugs in older adults is a decrease in serum albumin. Albumin concentrations decrease slightly with age in most elderly patients, although significant changes that may affect drug therapy may be seen in the chronically ill or malnourished elderly patient (Bourne, 2007). Albumin is the most common protein that binds to various acidic drugs. Significant decreases in albumin may result in a greater free concentration of highly protein-bound drugs. Box 4-3 lists some drugs that have significant protein binding, which may result in greater free concentrations when albumin is significantly reduced. Generally, drugs that are highly protein bound to albumin should be prescribed in reduced doses for patients with low serum albumin values (Bourne, 2007). A practical example of the clinical significance of this relationship can be described with the anticonvulsant phenytoin. In an elderly patient with a low serum albumin concentration (normal, 3.5 to 5 mg/dl), the phenytoin level reported from virtually all laboratories is the bound concentration. In a hypoalbuminemic individual, this value may appear normal or even subtherapeutic. This is because of the greater amounts of “free” or nonbound phenytoin that are getting into the tissue and acting at the receptor level but are not portrayed in the total serum level. The actual level may be much higher or even in the toxic range. Treatment decisions with older adults should not be based solely on drug levels. Treatment decisions must be based on consideration of both patient characteristics and drug levels.
Other protein changes also may have an influence on drug therapy. Patients with acute disease—such as myocardial infarction, respiratory distress, or infectious insult, for example—may experience increases in α1-glycoprotein, an acute phase reactant protein. This may result in the increased binding of weakly basic drugs, including propranolol or lidocaine, and a less-than-normal response to therapy. Data are lacking concerning the real significance of changes in α1-glycoprotein and drug therapy in the elderly (DiPiro et al, 2011).
Some drugs have an affinity for specific tissues. This affinity becomes useful when patients with certain infections are under treatment. For example, ciprofloxacin and tetracycline have an affinity for bone.
It is not generally necessary to calculate Vd when drugs are used. However, it is an important concept to understand when one is administering a drug, and it may influence the dosage or choice of a drug. Always consider where the drug will go and how much will get to the target organ or tissue.
The distribution of drugs from the bloodstream to the central nervous system is different than that through other cell membranes. The endothelial cells of the brain capillaries do not have intercellular pores and vesicles. Passive distribution of hydrophilic drugs is restricted. However, lipophilic drugs will easily pass the blood-brain barrier, limited only by cerebral blood flow (Brunton et al, 2010). Highly fat-soluble drugs also cross the blood-brain barrier easily and are likely to cause central nervous system side effects such as confusion and drowsiness.
Biotransformation (Metabolism)
Biotransformation is the chemical inactivation of a drug through conversion to a more water-soluble compound that can be excreted from the body. Biotransformation occurs primarily in the liver, but it also may be found in the lungs and in the GI tract. It involves two major steps in enzyme activity. Phase I makes the drug more hydrophilic through oxidation, reduction, or hydrolysis. Minor changes in the structure of the drug make it more hydrophilic but allow it to maintain all or part of its pharmacologic activity. The cytochrome P450 enzyme system is a part of phase I. Phase II is called glucuronidation. It involves conjugation, or attachment of particles to the molecule, making it a highly water-soluble substance with little or no pharmacologic activity (Brunton et al, 2010). If blood flow to the liver is decreased, drugs will be metabolized more slowly, leading to a longer duration of action.
Lipophilic drugs pass easily through membranes, including renal tubules, making them difficult to excrete. Biotransformation changes a lipophilic drug that is active and transforms it into a hydrophilic inactive compound that is readily excreted. However, metabolites occasionally have biologic activity or toxic properties (Figure 3-5).
Phase I: Oxidation or Reduction of Drugs
Phase I involves oxidation or reduction—hydrolysis reactions that make drugs more water soluble so they can be excreted. During this phase, perhaps as many as 40 different P450 enzymes present in the liver may be available to participate as catalysts in the oxidation of drugs. It appears that the metabolism of most drugs can be accounted for by a relatively small subset of these enzymes, with probably half attributed to CYP 3A4. The P450 enzyme system is a topic that is rapidly gaining expanded attention, and new information is becoming available constantly.
The cytochrome P450 enzyme system operates throughout the body. It is concentrated in the liver, intestine, and lungs. The P450 enzyme system resides in the ribosomes, which are sacs in the endoplasmic reticulum. It is named P450 because this is the length of the wave of light that these enzymes absorb. Chemically, the enzyme is a glycoprotein or a sugar plus a protein. Many of the proteins contain heme—hence the name chrome. This family of enzymes is divided into groups according to similarity. At least 40 major groups have been identified in humans so far. The major groups named 1, 2, 3, and 4 are known to be involved in drug interactions. These major groups are further divided into groups by their chemical structure, named A, B, and C. The A, B, and C groups are then divided into subgroups named 1, 2, 3, and so on. The groups that are most important in human drug interactions are CYP 2D6, CYP 3A3/4, CYP 1A2, CYP 2C9/10, and CYP 2C19. In the groups with 3/4 and 9/10, the two are so close in structure that they are difficult to differentiate and have very similar actions (Dresser et al, 2000; Lim et al, 2005; Zhou et al, 2004, 2005).
Advances in technology have resulted in an explosion of information concerning the cytochrome P450 isoenzymes and increased awareness of life-threatening interactions with such commonly prescribed drugs as cisapride and some antihistamines. Knowledge of the substrates, inhibitors, and inducers of these enzymes assists one in predicting clinically significant drug interactions.
The P450 enzyme system is not the same in every individual. Each person receives genetic material that determines individual variations in the enzyme system; this is called genetic polymorphism. Thus, individual differences and racial and gender differences occur. These are not yet well known or understood. Patients express their enzyme system in different ways. This explains why different patients react differently to a drug. For example, some patients metabolize codeine (CYP 2D6) quickly and need larger doses, whereas other patients metabolize it slowly and need less.
The essential facts to master about the P450 enzyme system are that six primary enzymes account for the metabolism of nearly all clinically important drugs, and two of these systems are critically important for drug metabolism (Table 3-1).
TABLE 3-1
Examples of Clinically Relevant Drugs Metabolized by Various CYP Enzymes
From Flockhart D: Cytochrome P450 drug-interaction table, Division of Clinical Pharmacology, Indiana University Department of Medicine, 2007 (Available at http://medicine.iupui.edu/flockhart/table.htm).
CYP 3A4 is an enzyme needed for metabolism of many drugs such as antihistamines, antibiotics, lipid-lowering drugs, antihypertensives, protease inhibitors, and azole antifungals. This system is used in the metabolism of approximately 50% of all clinically useful medications. These enzymes are the most abundant and clinically significant. (In CYP 3A4, the notation CYP indicates that the property is part of the cytochrome P450 system; 3 indicates the family; A indicates the subfamily; and 4 indicates that it is the fourth enzyme in that subsystem.)
The CYP 2D6 enzyme looks different and is different. It metabolizes selective serotonin reuptake inhibitors (SSRIs), pain relievers, β-blockers, and other drugs. This enzyme metabolizes about 30% of all clinically useful medications, is the second most abundant, and participates in converting codeine to morphine.
Of the other four enzymes, the most notable features of each are as follows:
A drug can be a substrate or one that is affected by alteration of its enzyme metabolism. A drug can also be the one that causes the alteration in the enzyme metabolism of another drug by being an inhibitor or an inducer. The cytochrome P450 enzyme system may speed up a reaction because it causes the drug to change to a more hydrophilic substance. Any drug that causes the enzyme to metabolize more slowly or decreases the capacity of the enzyme pathway is called an inhibitor. For example, if a patient on fluoxetine (Prozac) takes warfarin, the fluoxetine inhibits the P450 enzyme system from metabolizing warfarin and may produce an exaggerated therapeutic response (bleeding). A drug that causes the enzymes to metabolize the substrate more quickly is called an inducer. These types of drugs increase enzyme activity by increasing the number of CYP 450 enzymes. Any drug can be involved in this process in two ways. It can be the drug or substrate that is being acted upon, or it can be the inhibitor or inducer that is acting on the enzyme to increase or decrease enzyme conversion of the substrate drug into an inactive compound. The same drug can be both a substrate and an inducer or inhibitor (Brunton et al, 2010). For example, carbamazepine is an auto inducer—it induces its own metabolism. It is the enzyme system and not the drug that is being induced or inhibited (see Figure 3-5 to examine these relationships).
A drug can inhibit an enzyme pathway through two mechanisms. The first is competition, which is not usually a problem. If it occurs, it occurs immediately. Most inhibition is metabolic. The inhibitor drug decreases the production of the enzyme. It shrinks the enzyme pathway. This is not an immediate reaction; it may take anywhere from 24 hours up to a week to see the effect, depending on the half-life of the drug (Brunton et al, 2010). From a pharmacokinetic standpoint, the major effects of drug–drug interactions are understood in terms of causing a high or low plasma and tissue level of the drug.
Enzyme induction is much less common than inhibition. These drugs make the pathway work quickly, causing the substrate drug to be deactivated more rapidly. This will lower the level of the drug in the body. The clinically important inducers are anticonvulsants. All anticonvulsants should be considered as possible inducers. Evaluation of these drugs is particularly important when they are being added to a patient’s medication regimen.
In addition to cytochrome P450, oxidation of drugs and other xenobiotics can be mediated by non-P450 enzymes, the most significant of which are flavin monooxygenase, monoamine oxidase, alcohol dehydrogenase, aldehyde dehydrogenase, aldehyde oxidase, and xanthine oxidase. Drug oxidation catalyzed by some of these enzymes may often produce the same metabolites as those generated by P450; thus, drug interactions may be difficult to predict without a clear knowledge of the underlying enzymology. Although oxidation catalyzed by non-P450 enzymes can lead to drug inactivation, oxidation may be essential for the generation of active metabolites that create drug action (Brunton et al, 2010).
Phase II: Biotransformation
Phase II or biotransformation consists of conjugate reactions in which a compound is added to the drug. These reactions bind a chemical group to the drug compound via a covalent linkage. The chemical groups added to the drug are generally highly polar, or ionized. This makes them water soluble and generally inactive. However, a few of these conjugate compounds are active (Brunton et al, 2010).
First-Pass Effect: When a medication is taken orally, it passes from the intestine directly to the liver by way of the hepatic portal blood flow (via the portal vein). Many drugs, such as nitroglycerin and estrogen, are extensively metabolized to inert compounds when they first pass through the liver. Because of this, very large amounts of the drug must be given for a sufficient dose to remain after the first pass through the liver. This is known as the first-pass effect (Stringer, 2005). It is why these drugs are often given via an alternative route, such as sublingual or topical, to avoid the liver on the first pass. Alternative route dosing also makes it possible to give a smaller amount of the drug and have it be effective.
Prodrug: A prodrug is a chemical that is pharmacologically inactive. It is biotransformed into a biologically active metabolite in the body. The angiotensin-converting enzyme inhibitor enalapril is an example of a medication that must be transformed from a prodrug to the biologically active metabolite (Brunton et al, 2010). This activity may be reduced in congestive heart failure if the liver is congested, thus delaying treatment of the patient with heart failure.
Elimination
Elimination is the process by which drugs and their metabolites are removed from the body. The liver and the kidney are the two major organs responsible for elimination.
Most elimination occurs through excretion by the kidneys. The processes involved in renal elimination consist of glomerular filtration, tubular secretion, and partial reabsorption. In glomerular filtration, the drug enters the renal tubule by filtration. However, whether this occurs depends on the amount of protein binding because a drug does not filter into the tubule if it is bound to a protein. This action also depends on the rate of glomerular filtration, which in turn depends on the kidney function and cardiovascular status of the patient (Figure 3-6).
FIGURE 3-6 Drug elimination by the kidney. (Modified from Wong DL: Whaley and Wong’s nursing care of infants and children, ed 6, St Louis, 1998, Mosby.)
Active, carrier-mediated tubular secretion is responsible for adding some organic anion and cation molecules to the proximal renal tubule. The carrier systems are fairly nonselective and organic ions compete for transport. Thus, two drugs may compete for the same active transport carriers, and their excretion will be slowed (Brunton et al, 2010). Although it may be difficult to predict the result, a drug can be both secreted and actively reabsorbed. Penicillin is an important example of this process.
Once the drug has been excreted via active transport in the proximal tubule, weakly acidic or alkaline molecules may undergo passive reabsorption. How much is reabsorbed depends on the pH of the urine. Alkalinization of the urine will increase the excretion of acidic molecules. Acidification of the urine will increase the excretion of basic molecules. Clinical alteration of the pH of urine will hasten the excretion of some drugs, as in the case of drug poisoning or drug toxicity (DiPiro et al, 2011).
If the glomerular filtration rate in the kidneys is reduced, the drug remains in the blood longer. In patients with kidney damage, it is necessary to calculate an estimated creatinine clearance to modify the dosage of medication necessary. This is calculated with the following formula:
∗Lean body weight may reflect creatine production more accurately.
†In women, multiply the value × 0.85.
Drugs and their metabolites may be excreted through fecal and respiratory routes, through breast milk, and in other ways. The fecal route is often clinically significant. Drugs are metabolized in the liver, and the metabolites are excreted in the bile. These metabolites are reabsorbed into the blood and then are excreted in the urine or, less commonly, are simply excreted in the feces. Excretion from the liver into the bile is accomplished by active transport systems, which are limited in the quantity of metabolites they can excrete (Stringer, 2005). Different drugs may compete for transport. This competition may slow down the excretion of certain drugs; steroids provide an example of competition that slows excretion.
The respiratory route is an important route of excretion in anesthetic gases. Excretion in breast milk is important, not for the quantity excreted, but because of the effect it may have on a nursing infant. Other routes such as perspiration, saliva, tears, hair, and skin are not usually clinically significant.
Clearance is loosely defined as a measure of the rate at which the drug is removed from the body (Stringer, 2005). The body can eliminate the drug only if the drug is in contact with the eliminating organ. If a drug is being stored in adipose tissue, it cannot be cleared from the body. Thus, volume of distribution affects the clearance of a drug and hence the half-life.
Specifically, clearance is defined as the volume of plasma from which all drug is removed in a given time. It is measured as volume divided by time:
This formula yields milliliters of plasma that has had the drug removed from it in the specified amount of time (1 minute). Total body clearance is the sum of clearances from the various metabolizing and eliminating organs (Stringer, 2005).
Steady state means there is a stable concentration of the drug or the drug is being administered at the same rate at which it is being eliminated. This is fairly simple with a continuous infusion of drugs. With intermittent administration of medication, the frequency and amount of the drug must be adjusted to achieve steady state. Parkinson’s medications such as carbidopa/levodopa (Sinemet) often require adjustment for both the amount and the interval of dosing to achieve therapeutic levels without reaching toxic levels. Patients who are eliminating a drug more slowly than normal will need smaller doses than will patients who are eliminating the drug normally.
Plasma Concentration–Time Curve
The plasma concentration–time curve (Figure 3-7) illustrates what happens when a single dose of a drug is given. The time between when the drug is given and when it first takes effect is the latent period. Onset of action is the time it first takes effect. When the drug no longer has an effect, this is known as termination of action. The duration of action is the period of time during which the drug has its effect. The minimal effective concentration is the lowest level of concentration that produces the drug effect. The peak plasma level is the highest level the drug reaches. If the concentration is high enough to cause adverse drug reactions, this level is known as the toxic level. The therapeutic range is the area between the minimal effective concentration and the toxic concentration.
First-Order/Linear Kinetics
The concept of half-life is an important one to understand. The half-life of a drug is the amount of time required for the amount of drug in the body to decrease by one half. This is a significant factor in accumulation and elimination of drugs. It depends on clearance and volume of distribution. When a patient is given a drug on a regular schedule, the drug will continue to accumulate until steady state is achieved (Stringer, 2005) (Figure 3-8). The half-life of a drug listed in reference books is an approximation of what the most likely or usual half-life will be in a normal, healthy adult. However, much individual variation occurs and is based on factors that affect volume of distribution and elimination. Half-life is useful for estimating the amount of time it takes to reach a steady state of a drug after a dosage regimen is started. Steady state is reached after 4 to 5 half-lives, which represents 94% to 97% of the eventual steady state. Half-life also can be used to estimate the amount of time it takes to eliminate a drug from the body after it has been discontinued. Again, after 4 to 5 half-lives, about 94% to 97% of the drug will have been eliminated from the body (Brunton et al, 2010). Of course, there is always a difference between the absolute (or theoretical) steady state and what is seen in fact for each patient.
FIGURE 3-8 Drug accumulation time to steady state. The line shows concentrations of drugs in the body. (Modified from Brunton LL, Chabner B, Knollman B, editors: Goodman & Gilman’s the pharmacological basis of therapeutics, ed 12, New York, 2010, McGraw-Hill.)
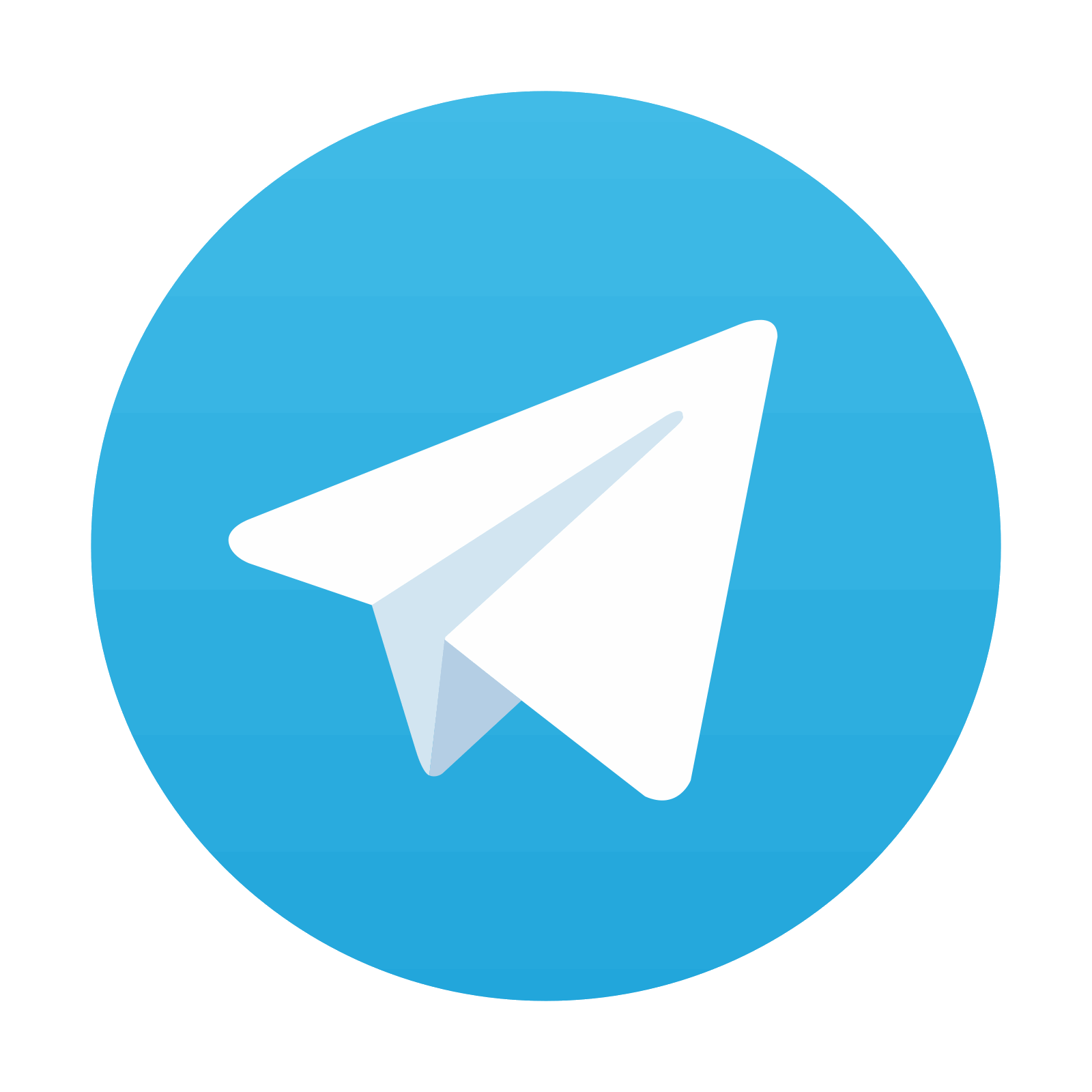
Stay updated, free articles. Join our Telegram channel
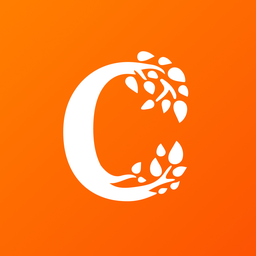
Full access? Get Clinical Tree
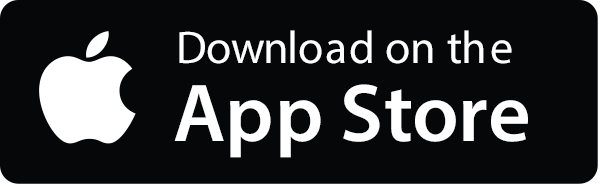
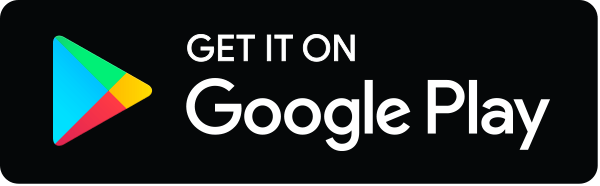