4 Fixation of tissues
Introduction
Although each fixative has advantages, they all have many disadvantages. These include molecular loss from ‘fixed’ tissues, swelling or shrinkage of tissues during the process, variations in the quality of histochemical and immunohistochemical staining, the ability to perform biochemical analysis accurately, and varying capabilities to maintain the structures of cellular organelles. One of the major problems with fixation using formaldehyde has been the loss of antigen immunorecognition due to that type of fixation combined with processing the tissue to paraffin wax (Eltoum et al. 2001a, 2001b). However, from a clinical perspective the advent of heat-induced epitope retrieval methods, instigated in the early 1990s, have overcome many of these limitations (Shi et al. 1991). Similarly, the analysis of mRNA and DNA from formalin-fixed, paraffin-embedded tissue has been problematic (Grizzle et al. 2001; Jewell et al. 2002; Steg et al. 2006; Lykidis et al. 2007). All widely used fixatives are selected by compromise; their good aspects are balanced against less desirable features. This chapter discusses the basics of fixation, the advantages and disadvantages of specific fixatives, and provides some of the formulae for specific fixatives currently used in pathology, histology, and anatomy.
The major objective of fixation in pathology is to maintain clear and consistent morphological features (Eltoum et al. 2001a, 2001b; Grizzle et al. 2001). The development of specific fixatives usually has been empirical, and much of the understanding of the mechanisms of fixation has been based upon information obtained from leather tanning and vaccine production. In order to visualize the microanatomy of a tissue, its stained sections must maintain the original microscopic relationships among cells, cellular components (e.g. the cytoplasm and nuclei), and extracellular material with little disruption of the organization of the tissue, and must maintain the tissue’s local chemical composition. Many tissue components are soluble in aqueous acid or other liquid environments, and a reliable view of the microanatomy and microenvironment of these tissues requires that the soluble components are not lost during fixation and tissue processing. Minimizing the loss of cellular components, including proteins, peptides, mRNA, DNA, and lipids, prevents the destruction of macromolecular structures such as cytoplasmic membranes, smooth endoplasmic reticulum, rough endoplasmic reticulum, nuclear membranes, lysosomes, and mitochondria. Each fixative, combined with the tissue processing protocol, maintains some molecular and macromolecular aspects of the tissue better than other fixative/processing combinations. For example, if soluble components are lost from the cytoplasm of cells, the color of the cytoplasm on hematoxylin and eosin (H&E) staining will be reduced or modified and aspects of the appearance of the microanatomy of the tissue, e.g. mitochondria, will be lost or damaged. Similarly, immunohistochemical evaluations of structure and function may be reduced or lost.
Almost any method of fixation induces shrinkage/swelling, hardening of tissues and color variations in various histochemical stains (Sheehan & Hrapchak 1980; Horobin 1982; Fox et al. 1985; Carson 1990; Kiernan 1999; O’Leary & Mason 2004). Various methods of fixation always produce some artifacts in the appearance of tissue on staining; however, for diagnostic pathology it is important that such artifacts are consistent.
The fixative acts by minimizing the loss or enzymatic destruction of cellular and extracellular molecules, maintaining macromolecular structures and protecting tissues from destruction by microorganisms. This results in one view of a dynamically changing, viable tissue (Grizzle et al. 2001). A fixative should also prevent the subsequent breakdown of the tissue or molecular features by enzymatic activity and/or by microorganisms during long-term storage, because diagnostic/therapeutic tissues removed from patients are important resources which may be re-analyzed in the future.
A fixative not only interacts initially with the tissue in its aqueous environment but also, subsequently, the unreacted fixative and the chemical modifications induced by the fixative continue to react. Fixation interacts with all phases of processing and staining from dehydration to staining of tissue sections using histochemical, enzymatic or immunohistochemical stains (Eltoum et al. 2001b; Rait et al. 2004). A stained tissue section produced after specific fixation combined with tissue processing produces a compromise in the picture that is formed of one or more features of the original living tissue. To date, a universal or ideal fixative has not been identified. Fixatives are therefore selected based on their ability to produce a final product needed to demonstrate a specific feature of a specific tissue (Grizzle et al. 2001). In diagnostic pathology, the fixative of choice for most pathologists has been 10% neutral buffered formalin (Grizzle et al. 2001).
The most important characteristic of a fixative is to support high quality and consistent staining with H&E, both initially and after storage of the paraffin blocks for at least a decade. The fixative must have the ability to prevent short- and long-term destruction of the micro-architecture of the tissue by stopping the activity of catabolic enzymes and hence autolysis, minimizing the diffusion of soluble molecules from their original locations. Another important characteristic of a good fixative, which helps maintain tissue and cellular integrity, is the inanimation of infectious agents, which helps maintain tissue and cellular integrity. It is also important to have good toxicological and flammability profiles that permit the safe use of the fixative (Grizzle & Fredenburgh 2005). The advent of new biological methods, increased understanding of the human genome, and the need to rapidly evaluate the biology of disease processes, means that fixatives should also permit the recovery of macromolecules including proteins, mRNA, and DNA without extensive biochemical modifications from fixed and paraffin-embedded tissues.
Other important characteristics of an ideal fixative include being useful for a wide variety of tissues, including fatty, lymphoid, and neural tissues. It should preserve small and large specimens and support histochemical, immunohistochemical, in situ hybridization and other specialized procedures. It should penetrate and fix tissues rapidly, have a shelf life of at least one year, and be compatible with modern automated tissue processors. The fixative should be readily disposable or recyclable and support long-term tissue storage giving excellent microtomy of paraffin blocks, and should be cost- effective (Dapson 1993).
Types of fixation
Fixation of tissues can be accomplished by physical and/or chemical methods. Physical methods such as heating, microwaving, and freeze-drying are independent processes and not used commonly in the routine practice of medical or veterinary pathology, anatomy, and histology, except for the use of dry heat fixation of microorganisms prior to Gram staining. Most methods of fixation used in processing of tissue for histopathological diagnoses rely on chemical fixation carried out by liquid fixatives. Reproducibility over time of the microscopic appearances of tissues after H&E staining is the prime requirement of the fixatives used for diagnostic pathology. Methods of fixation used in research protocols may be more varied, including fixation using vapors and fixation of whole animals by perfusing the animal’s vascular system with a fixative (Eltoum et al. 2001a, 2001b).
Several chemicals or their combinations can act as good fixatives, and accomplish many of the stated goals of fixation. Some fixatives add covalent reactive groups which may induce cross-links between proteins, individual protein moieties, within nucleic acids, and between nucleic acids and proteins (Horobin 1982; Eltoum et al. 2001a, 2001b; Rait et al. 2004, 2005). The best examples of such ‘cross-linking fixatives’ are formaldehyde and glutaraldehyde. Another approach to fixation is the use of agents that remove free water from tissues and hence precipitate and coagulate proteins; examples of these dehydrants include ethanol, methanol, and acetone. These agents denature proteins by breaking the hydrophobic bonds which are responsible for the tertiary structure of proteins. Other fixatives, such as acetic acid, trichloroacetic acid, mercuric chloride, and zinc acetate, act by denaturing proteins and nucleic acids through changes in pH or via salt formation. Some fixatives are mixtures of reagents and are referred to as compound fixatives, e.g. alcoholic formalin acts to fix tissues by adding covalent hydroxymethyl groups and cross-links as well as by coagulation and dehydration.
Physical methods of fixation
Microwave fixation
Microwave heating speeds fixation and can reduce times for fixation of some gross specimens and histological sections from more than 12 hours to less than 20 minutes (Anonymous 2001; Kok & Boon 2003; Leong 2005). Microwaving tissue in formalin results in the production of large amounts of dangerous vapors, so in the absence of a hood for fixation, or a microwave processing system designed to handle these vapors, this may cause safety problems. Recently, commercial glyoxal-based fixatives which do not form vapors when heated at 55°C have been introduced as an efficient method of microwave fixation.
Freeze-drying and freeze substitution
Freeze-drying is a useful technique for studying soluble materials and small molecules; tissues are cut into thin sections, immersed in liquid nitrogen, and the water is removed in a vacuum chamber at −40°C. The tissue can be post-fixed with formaldehyde vapor. In substitution, specimens are immersed in fixatives at −40°C, such as acetone or alcohol, which slowly remove water through dissolution of ice crystals, and the proteins are not denatured; bringing the temperature gradually to 4°C will complete the fixation process (Pearse 1980). These methods of fixation are used primarily in the research environment and are rarely used in the clinical laboratory setting.
Chemical fixation
Chemical fixation utilizes organic or non-organic solutions to maintain adequate morphological preservation. Chemical fixatives can be considered as members of three major categories: coagulant, cross-linking, and compound fixatives (Baker, 1958).
Dehydrant coagulant fixatives
The most commonly used coagulating fixatives are alcohols (e.g. ethanol, methanol) and acetone. Methanol is closer to the structure of water than ethanol. Ethanol therefore competes more strongly than methanol in the interaction with hydrophobic areas of molecules; thus, coagulant fixation begins at a concentration of 50–60% for ethanol but requires a concentration of 80% or more for methanol (Lillie & Fullmer 1976). Removal and replacement of free water from tissue by any of these agents has several potential effects on proteins within the tissue. Water molecules surround hydrophobic areas of proteins and, by repulsion, force hydrophobic chemical groups into closer contact with each other and hence stabilize hydrophobic bonding. By removing water, the opposite principle weakens hydrophobic bonding. Similarly, molecules of water participate in hydrogen bonding in hydrophilic areas of proteins; so removal of water destabilizes this hydrogen bonding. Together, these changes act to disrupt the tertiary structure of proteins. In addition, with the water removed, the structure of the protein may become partially reversed, with hydrophobic groups moving to the outside surface of the protein. Once the tertiary structure of a soluble protein has been modified, the rate of reversal to a more ordered soluble state is slow and most proteins after coagulation remain insoluble even if returned to an aqueous environment.
Disruption of the tertiary structure of proteins, i.e. denaturation, changes their physical properties, potentially causing insolubility and loss of function. Even though most proteins become less soluble in organic environments, up to 13% of protein may be lost, for example with acetone fixation (Horobin 1982). Factors that influence the solubility of macromolecules include:
1. Temperature, pressure, and pH.
2. Ionic strength of the solute.
3. The salting-in constant, which expresses the contribution of the electrostatic interactions.
4. The salting-in and salting-out interactions.
5. The type(s) of denaturing reagent(s) (Herskovits et al. 1970; Horobin 1982; Papanikolau & Kokkinidis 1997; Bhakuni 1998).
Alcohol denatures protein differently, depending on the choice and concentration of alcohol, the presence of organic and non-organic substances, and the pH and temperature of fixation. For example, ethanol denatures proteins > phenols > water and polyhydric alcohols > monocarboxylic acids > dicarboxylic acids (Bhakuni 1998).
Other types of coagulant fixative
Acidic coagulants such as picric acid and trichloroacetic acid change the charges on the ionizable side chains, e.g. (NH2 → NH3+) and (COO− → COOH), of proteins and disrupt electrostatic and hydrogen bonding. These acids also may insert a lipophilic anion into a hydrophilic region and hence disrupt the tertiary structures of proteins (Horobin 1982). Acetic acid coagulates nucleic acids but does not fix or precipitate proteins; it is therefore added to other fixatives to prevent the loss of nucleic acids. Trichloroacetic acid (Cl3CCOOH) can penetrate hydrophobic domains of proteins and the anion produced (
C
COO−) reacts with charged amine groups. This interaction precipitates proteins and extracts nucleic acids. Picric acid or trinitrophenol slightly dissolves in water to form a weak acid solution (pH 2.0). In reactions, it forms salts with basic groups of proteins, causing the proteins to coagulate. If the solution is neutralized, precipitated protein may redissolve. Picric acid fixation produces brighter staining, but the low pH solutions of picric acid may cause hydrolysis and loss of nucleic acids
Non-coagulant cross-linking fixatives
Several chemicals were selected as fixatives secondary to their potential actions of forming cross-links within and between proteins and nucleic acids as well as between nucleic acids and proteins. Cross-linking may not be a major mechanism at current short times of fixation, and therefore ‘covalent additive fixatives’ may be a better name for this group. Examples include formaldehyde, glutaraldehyde, and other aldehydes, e.g. chloral hydrate and glyoxal, metal salts such as mercuric and zinc chloride, and other metallic compounds such as osmium tetroxide. Aldehyde groups are chemically and biologically reactive and are responsible for many histochemical reactions, e.g. free aldehyde groups may be responsible for argentaffin reactions (Papanikolau & Kokkinidis 1997).
Formaldehyde fixation
Formaldehyde in its 10% neutral buffered form (NBF) is the most common fixative used in diagnostic pathology. Pure formaldehyde is a vapor that, when completely dissolved in water, forms a solution containing 37–40% formaldehyde; this aqueous solution is known as ‘formalin’. The usual ‘10% formalin’ used in fixation of tissues is a 10% solution of formalin; i.e., it contains about 4% weight to volume of formaldehyde. The reactions of formaldehyde with macromolecules are numerous and complex. Fraenkel-Conrat and his colleagues, using simple chemistry, meticulously identified most of the reactions of formaldehyde with amino acids and proteins (French & Edsall 1945; Fraenkel-Conrat & Olcott 1948a, 1948b; Fraenkel-Conrat & Mecham 1949). In an aqueous solution formaldehyde forms methylene hydrate, a methylene glycol as the first step in fixation (Singer 1962).
Formaldehyde also reacts with nuclear proteins and nucleic acids (Kok & Boon 2003; Leong 2005). It penetrates between nucleic acids and proteins and stabilizes the nucleic acid-protein shell, and it also modifies nucleotides by reacting with free amino groups, as it does with proteins. In naked and free DNA, the cross-linking reactions are believed to start at adenine-thymidine (AT)-rich regions and cross-linking increases with increasing temperature (McGhee & von Hippel 1975a, 1975b, 1977a, 1977b). Formaldehyde reacts with CC and
SH bonds in unsaturated lipids, but does not interact with carbohydrates (French & Edsall 1945; Hayat 1981).
The side chains of peptides or proteins that are most reactive with methylene hydrate, and hence have the highest affinity for formaldehyde, include lysine, cysteine, histidine, arginine, tyrosine, and reactive hydroxyl groups of serine and threonine (see Table 4.1) (Means & Feeney 1995).
Gustavson (1956) reported that one of the most important cross-links in ‘over-fixation’, i.e. in tanning, is that between lysine and the amide group of the protein backbone. Due to the shorter fixation times of current diagnostic pathological and biological applications, cross-linking reactions with the protein backbone are unlikely to occur (French & Edsall 1945; Fraenkel-Conrat et al. 1945, 1947; Fraenkel-Conrat & Olcott 1948a, 1948b; Fraenkel-Conrat & Mecham 1949; Gustavson 1956).
Reversibility of formaldehyde-macromolecular reactions
Washing for 24 hours removes about half of reactive groups, and 4 weeks of washing removes up to 90% (Helander 1994). This suggests that actual cross-linking is a relatively slow process, so, in the rapid fixation used in diagnostic pathology, most ‘fixation’ with formaldehyde prior to tissue processing stops with the formation of reactive hydroxymethyl groups.
For long-term storage in formalin, the reactive groups may be oxidized to the more stable groups (e.g. acids NH
COOH) which are not easily removed by washing in water or alcohol. Thus, following fixation, returning the specimen to water or alcohol further reduces the fixation of the specimen, because the reactive groups produced by the initial reaction with formalin may reverse and be removed. Although it was initially thought that cross-linking was most important in the fixation of tissue for biological uses (based on the limited number of cross-links over short periods of fixation), it is likely that formation of these hydroxymethyl groups actually denatures macromolecules and renders them insoluble. As these washing experiments have not been reproduced, the actual mechanisms and their importance to fixation by formaldehyde are uncertain. As well as simple washing under running water, over-fixation of tissue may be partially corrected by soaking the tissue in concentrated ammonia plus 20% chloral hydrate (Lhotka & Ferreira 1949). Fraenkel-Conrat and his colleagues frequently noted that the addition and condensation reactions of formaldehyde with amino acids and proteins were unstable and could be reversed easily by dilution or dialysis (Fraenkel-Conrat et al. 1945, 1947; Fraenkel-Conrat & Olcott 1948a, 1948b; Fraenkel-Conrat & Mecham 1949).
The principal type of cross-link in short-term fixation is thought to be between the hydroxymethyl group on a lysine side chain and arginine (through secondary amino groups), asparagine, glutamine (through secondary amide groups), or tyrosine (through hydroxyl group) (Tome et al. 1990). For example, a lysine methyl hydroxyl amine group can react with an arginine group to form a lysineCH2–arginine cross-link; similarly, a tyrosine methyl hydroxyl amine group can bind with a cysteine group to form a tyrosine
CH2–cysteine cross-link. Each of these cross-links between macromolecules has a different degree of stability, which can be modified by the temperature, pH, and type of the environment surrounding and permeating the tissue (Eltoum et al. 2001b). The time to saturation of human and animal tissues with active groups by formalin is about 24 hours, but cross-linking may continue for many weeks (Helander 1994).
When formaldehyde dissolves in an unbuffered aqueous solution, it forms an acid solution (pH 5.0–5.5) because 5–10% of commercially available formaldehyde is formic acid. Acid formalin may react more slowly with proteins than NBF because amine groups become charged (e.g. N+H3). In solution, this requires a much lower pH than 5.5. However, the requirement for a lower pH to produce
N+H3 groups may not be equivalent to that required in peptides. Acid formalin also preserves immunorecognition much better than NBF (Arnold et al. 1996), and indeed the success of Taylor in the early days of immunocytochemistry to demonstrate immunoglobulins in paraffin-processed tissue sections, most probably relied on the fixation of the tissues in acid formalin (Taylor et al. 1974). The disadvantage of using acid formalin for fixation is the formation of a brown-black pigment with degraded hemoglobulin. This heme-related pigment, which forms in tissue, is usually not a great problem unless patients have a blood abnormality (e.g. sickle cell disease, malaria).
Formaldehyde primarily preserves peptide-protein bonds and the general structure of cellular organelles. It can interact with nucleic acids but has little effect on carbohydrates and preserves lipids if the solutions contain calcium (Bayliss High & Lake 1996).
Glutaraldehyde fixation
Less is known about glutaraldehyde’s biological reactions and effects compared to formaldehyde, as it has not been used as widely in biological applications. Glutaraldehyde is a bifunctional aldehyde that probably combines with the same reactive groups as does formaldehyde. In aqueous solutions glutaraldehyde polymerizes, forming cyclic and oligomeric compounds (Hopwood 1985), and it is also oxidized to glutaric acid. To aid in stability, it requires storage at 4°C and at a pH of around 5 (Hopwood 1969).
Unlike formaldehyde, glutaraldehyde has an aldehyde group on both ends of the molecule. With each reaction of the first group, an unreacted aldehyde group may be introduced into the protein and these aldehyde groups can act to further cross-link the protein. Alternatively, the aldehyde groups may react with a wide range of other histochemical targets, including antibodies, enzymes, or proteins. The reaction of glutaraldehyde with an isolated protein, such as bovine serum albumin, is fastest at pH 6–7, and is faster (Habeeb 1966), and results in more cross-linking than formaldehyde (Habeeb 1966; Hopwood 1969). Cross-linking is irreversible and withstands acids, urea, semicarbazide, and heat (Hayat 1981). Like formaldehyde, reactions with lysine are the most important for forming cross-links.
Extensive cross-linking by glutaraldehyde results in better preservation of ultrastructure, but this method of fixation negatively affects immunohistochemical methods and slows the penetration by the fixative. Thus, any tissue fixed in glutaraldehyde must be small (0.5 mm maximum) and, unless the aldehyde groups are blocked, increased background staining will result if several histochemical methods are used (Grizzle 1996a). Glutaraldehyde does not react with carbohydrates or lipids unless they contain free amino groups as are found in some phospholipids (Hayat 1981). At room temperature glutaraldehyde does not cross-link nucleic acids in the absence of nucleohistones but it may react with nucleic acids at or above 45°C (Hayat 1981).
Osmium tetroxide fixation
Osmium tetroxide (OsO4), a toxic solid, is soluble in water as well as non-polar solvents and can react with hydrophilic and hydrophobic sites including the side chains of proteins, potentially causing cross-linking (Hopwood et al. 1990). The reactive sites include sulfydryl, disulfide, phenolic, hydroxyl, carboxyl, amide, and heterocyclic groups. Osmium tetroxide is known to interact with nucleic acids, specifically with the 2,3-glycol moiety in terminal ribose groups and the 5,6 double bonds of thymine residues. Nuclei fixed in OsO4 and dehydrated with alcohol may show prominent clumping of DNA. This unacceptable artifact can be prevented by pre-fixation with potassium permanganate (KMnO4), post-fixation with uranyl acetate, or by adding calcium ions and tryptophan during fixation (Hayat 1981). The reaction of OsO4 with carbohydrates is uncertain (Hayat 1981). Large proportions of proteins and carbohydrates are lost from tissues during osmium fixation; some of this may be due to the superficial limited penetration of OsO4 (i.e. <1 mm) into tissues or its slow rates of reaction. In electron microscopy, this loss is minimized by initial fixation of tissue in glutaraldehyde.
In addition to its use as a secondary fixative for electron microscope examinations, OsO4 can also be used to stain lipids in frozen sections. Osmium tetroxide fixation causes tissue swelling which is reversed during dehydration steps. Swelling can also be minimized by adding calcium or sodium chloride to osmium-containing fixatives (Hayat 1981).
Mercuric chloride
Historically, mercuric chloride was greatly favored for its qualities of enhancing the staining properties of tissues, particularly for trichrome stains. However, it is now rarely used in the clinical laboratory due to the health and safety issues involved with the use of a mercury-containing fixative, and also due to the reduced reliance on ‘special stains’. A further major disadvantage of mercuric chloride fixation is the inevitable formation of deposits of intensely black precipitates of mercuric pigment in the tissues. This subsequently gives them inferior value for immunohistochemical and molecular studies. In recently fixed tissues, these precipitates can be readily removed by a Lugol’s iodine step in the staining procedure, followed by bleaching of the section in sodium hypochlorite solution (Hypo). However, this is not effective on mercuric chloride fixed tissues which have been stored for a number of years as paraffin blocks. In these tissues, retrospective analysis by immunohistochemistry and molecular techniques becomes unreliable due to the formation of much larger aggregates of mercuric pigment which cannot be removed subsequently by Lugol’s iodine. The chemistry of fixation using mercuric chloride is not well understood. It is, however, known that mercuric chloride reacts with ammonium salts, amines, amides, amino acids, and sulfydryl groups, and hardens tissues. It is especially reactive with cysteine, forming a dimercaptide (Hopwood 2002) and acidifying the solution:
If only one cysteine is present, a reactive group of RS
Hg
Cl is likely.
Mercury-based fixatives are toxic and should be handled with care. They should not be allowed to come into contact with metal, and should be dissolved in distilled water to prevent the precipitation of mercury salts. Mercury-containing chemicals are an environmental disposal problem. These fixatives penetrate slowly, so specimens must be thin, and mercury and acid formaldehyde hematein pigments may deposit in tissue after fixation. Mercury fixatives (Hopwood 1973) are no longer used routinely except by some laboratories for fixing hematopoietic tissues (especially B5). A potential replacement for mercuric chloride is zinc sulfate. Special formulations of zinc sulfate in formaldehyde replacing mercuric chloride in B5 may give better nuclear detail than formaldehyde alone and improve tissue penetration (Carson 1990).
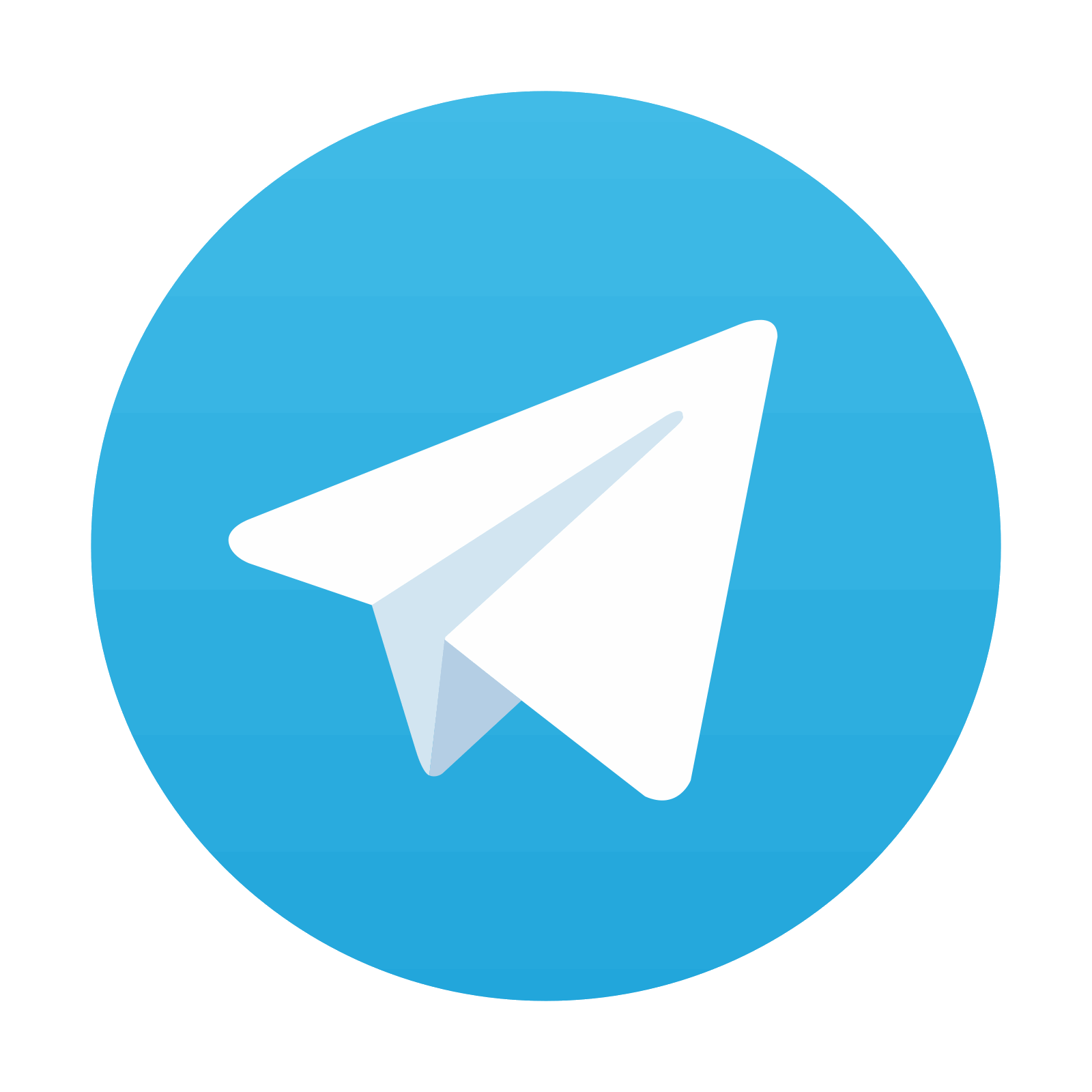
Stay updated, free articles. Join our Telegram channel
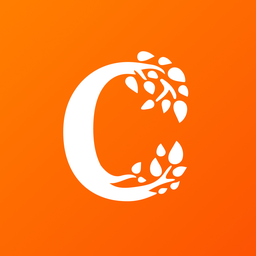
Full access? Get Clinical Tree
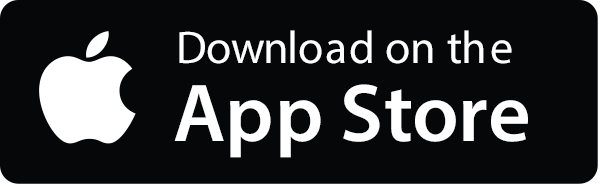
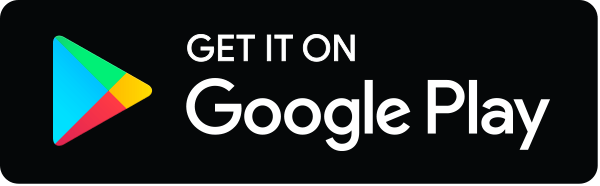