Figure 99-1. Fetal circulation. (From Rosdahl CB, Kowalski MT, eds. Textbook of Basic Nursing. Philadelphia, PA: Wolters Kluwer Health; 2012.)
Transition to Postnatal Life
3 One of the most critical and fascinating periods in human physiology is the changes which occur, usually in a matter of minutes, which allows a human to transition from surviving in the uterine environment to the external environment. Around the time of birth, epithelial cells cease production of lung fluid and begin to actively absorb it back into the fetal circulation. This process is facilitated by active sodium transport which is stimulated by thyroid hormone, glucocorticoids, and epinephrine.1 During the first breaths, pulmonary arterial pO2 increase and pCO2 decrease resulting in pulmonary vascular dilation, decreased pulmonary vascular resistance, and constriction of the DA. Although pulmonary vascular resistance decreases dramatically at birth, pulmonary artery pressure, pulmonary blood flow, and pulmonary vascular resistance decrease gradually over the first few weeks of life. The increased pulmonary blood flow results in an increase in left atrial pressure and as a result, the flap-like foramen ovale closes in response to higher left atrial pressures relative to right atrial pressure. The RV compliance gradually increases as well, and the resultant change in the differences in ventricular compliance allows the flap of the foramen ovale to gradually close over in the first few days to week of life, minimizing mixing, so that the outputs of the left and right ventricles become equivalent. The DA closes in the first 24 to 48 hours of life as a result of changes in direction of flow, contractile elements, an increase in pO2, a drop in PGE synthesized by the placenta, and other humoral factors. Removal of the placenta results in a decrease in circulating prostaglandin levels and increasing pO2 levels are a stimulus for ductal closure. When the foramen ovale or DA does not close, they are referred as patent foramen ovale (PFO) and patent ductus arteriosus (PDA). Certain clinical conditions may contribute to the persistence of fetal circulation or to the reappearance of fetal shunts under stress. Prematurity and critical illness are both associated with persistent fetal circulation. Under these conditions, the RV continues to contribute a large portion of the CO and the postductal saturations will be significantly lower than the preductal saturations. In premature infants, the transition may be much slower and the DA may not close despite the usual changes in systemic and pulmonary vascular resistance and the subsequent reversal of flow. Cessation of umbilical blood flow results in closure of the ductus venosus and increase in systemic vascular resistance. This increases the left-sided heart pressures and closes the foramen ovale. With these changes, the transition to postnatal circulation is complete and the process of postnatal pulmonary dependence is initiated.
Figure 99-2. The oxygen dissociation curve shows the percent saturation of Hgb at various partial pressure of oxygen and demonstrates the equilibrium between oxyhemoglobin and deoxyhemoglobin. The sigmoidal shape is a result of the cooperative binding between the four hemoglobin polypeptide chains. An important point is the P50 which is the partial pressure of oxygen at which erythrocytes are 50% saturated with oxygen. When oxygen partial pressure is high, such as in the lungs, hemoglobin binds oxygen with increased affinity. When the partial pressure of oxygen decreases such as in the peripheral tissues, oxygen is preferentially unloaded. Multiple factors including acid–base, temperature, and 2,3 DPG affect hemoglobins affinity for oxygen shifting the curve resulting in more or less unloading of oxygen at a given partial pressure.
In order for gas exchange to occur in the developing lung, there needs to be intimate contact between atmospheric oxygen and capillary blood flow. This requires adequate alveolar ventilation and pulmonary blood flow. The neonate has a number of physiologic mechanisms which allow for matching alveolar ventilation (V) and pulmonary perfusion (Q) to optimize gas exchange. To accomplish this, an adequate alveolar gas volume and FRC must be established shortly after birth and sustained. Once the FRC is established, this serves as an intrapulmonary pool of oxygen. However, newborns are at increased risk for hypoxemia due to lower than adult pO2 with less intravascular reserve, FRC closer to airway closure, and high metabolic demand in infancy resulting in quicker depletion of oxygen stores.
At birth, the oxygen demand in the infant increases in most species by 100% to 150%. Consequently, fetal hemoglobin with its increased oxygen affinity which was adequate for a fetus does not provide enough oxygen diffusion and delivery in the neonate. Postnatally, the infant’s affinity decreases rapidly and reaches normal adult values by 4 to 6 months which corresponds to the amount of time necessary for the replacement of fetal hemoglobin by adult hemoglobin.13 The amount of HgbF decreases from around 75% to approximately 2% during the first year of life. Until this occurs, other mechanisms help the neonate to cope with the ex utero environment. One of the most important is an increase in the concentration of 2,3 DPG during the first few days of life. At birth, its level is near an adult’s level of 5.43 ± 1.04 and increases to 6.58 ± 1.00. This increase has a small direct effect on fetal hemoglobin, but also lowers the intracellular pH and thus decreases hemoglobin’s affinity for oxygen.14
Surfactant
4 While many factors play a critical role in allowing for a smooth transition, one of the most clinically relevant pieces is the role surfactant in neonatal pulmonary mechanics and gas exchange. In pulmonary physiology, an important concept is surface tension and its effects on the pressure drop across an interface separating two phases of matter which was defined in the early 1800s by Young and Laplace.15 The pressure drop necessary to maintain or inflate air sacs of a given size is proportional to its surface tension and inversely proportional to its radius. The work of breathing is consequently directly proportional to the surface tension. During development, surfactant production increases as type II pneumocytes mature and alveoli increase in size. Surfactant is a mixture of phospholipids, neutral lipids, and specific proteins that, by virtue of their amphipathic nature, decrease surface tension, stabilize small alveoli, and improve overall alveolar inflation. Surfactant reduces the hydrostatic driving force for pulmonary edema and decreases work of breathing. When deficient, it can lead to severe respiratory failure. Exogenous administration has been proven effective in prophylactic or rescue treatment for respiratory distress syndrome (RDS) in premature infants with a 40% reduction in death and a 30% to 50% reduction in the odds of pulmonary air leaks.16 Additionally, vitamin A supplementation may reduce the risk of chronic lung disease (CLD) in extremely low–birth-weight infants and was recommended in infants under 1,000-g birth weight though further studies have failed to confirm the finding.17,18
Fetal Interventions
Fetal surgery has emerged as an independent subspecialty at the intersection of pediatric surgery, maternal fetal medicine, and neonatology. It is a field that has arisen from clinical necessity. Pediatric surgeons, maternal fetal medicine specialists, and neonatologists became frustrated with the management of a number of congenital structural anomalies in which the baby died or suffered severe life-long disability despite all efforts at postnatal treatment. With the advent of prenatal ultrasound in the 1970s, many conditions were diagnosed before birth. Fetuses were followed and the prenatal natural history was elucidated. It became clear that a component of organ failure was acquired because of ongoing alterations in fetal development caused by the anomaly. Thus, it made sense that fetal interventions may be of benefit – to correct the pathophysiology to restore normal fetal development in the hope of improving survival and decreasing morbidity.
Criteria that must be met to consider fetal surgery include: (a) accurate diagnosis of the condition and any associated anomalies that may have an impact on outcome; (b) reliable prediction of which individual fetuses will die or suffer serious long-term morbidity without fetal intervention; and (c) demonstration of improved fetal outcome with minimal maternal risk.
Fetal imaging is a critical component of the decision making process in fetal surgery. Prenatal detection and serial ultrasonographic evaluation of these disorders have enhanced our understanding of their natural history and pathophysiology, and have significantly improved perinatal management. Fetal conditions that may benefit from in utero intervention by a pediatric surgeon include myelomeningocele, twin to twin transfusion syndrome, sacrococcygeal teratoma (SCT), congenital diaphragmatic hernia (CDH), and congenital lung lesions (CLLs).
Sacrococcygeal Teratoma
The evolution of high-output cardiac failure before the development of placentomegaly and hydrops in a fetus with SCT is the sole indication for fetal surgical intervention. Furthermore, SCT may lead to maternal mirror syndrome (Ballantine syndrome).19 Fetal echocardiography and Doppler ultrasound measurements are important in the diagnostic assessment and follow-up of fetuses with these conditions. Increased aortic velocity, increased combined CO, increased cardiac-to-thoracic ratio, a dilated inferior vena cava, or reversed end-diastolic umbilical blood flow appears to be sensitive early predictors of impending hydrops and fetal demise.20,21 For fetuses under 28 weeks estimated gestational age (EGA), open fetal surgery and resection is the treatment of choice.
Congenital Diaphragmatic Hernia
At present, the role of fetal intervention in the management of fetuses with CDH is not clear. The pathophysiology of CDH involves both pulmonary hypoplasia and pulmonary hypertension.
Although the cause of CDH remains unknown, the consequences to pulmonary development and function are well known. If the pleuroperitoneal canal has not closed when the midgut returns to the abdomen by weeks 9 to 10, the abdominal viscera herniates into the chest cavity. This affects both ipsilateral and contralateral lung development, with hypoplasia more severe on the ipsilateral side. The herniation occurs during the period of bronchial subdivisions. This stage becomes compromised with the major bronchial buds present but the number of bronchial branches significantly reduced in both ipsilateral and contralateral pulmonary specimens.22 Consequently, alveolization is severely affected with significantly fewer normal alveoli at birth.23
Pulmonary vascular beds are also distinctly abnormal with a reduction in the total number of arterial branches in both the ipsilateral and contralateral pulmonary parenchyma with significant adventitial and medial wall thickening noted with a resultant increased risk for fixed and intractable pulmonary hypertension.1 With the institution of breathing, the pulmonary vascular resistance normally decreases allowing for increased pulmonary blood flow. If the pulmonary vascular resistance remains high after the transition to postnatal life, right to left shunting of blood can occur with delivery of unsaturated blood to the systemic circulation with resultant hypoxia. The hypoxia can lead to further increases in pulmonary vascular resistance and compromises to pulmonary flow while increasing right-to-left blood shunting leading to severe and progressive respiratory failure. Postnatally, there is also a failure of the normal arterial remodeling maintaining an abnormally high vascular resistance that is only partially reversible by treatment interventions.24
Based on both experimental work in the laboratory and an understanding of congenital high airway obstruction, the technique of in utero tracheal occlusion was developed to prevent egress of lung fluid and enhance lung growth.25 Although early results did not appear superior to postnatal management,26 Deprest and his group refined the technique and showed promising results compared to historical controls.27 This innovative strategy is being tested in a multicenter randomized clinical trial.28
Congenital Lung Lesions
While CDH results in hypoplasia of the lung due to a disruption of early lung development, other CLLs including congenital pulmonary airway malformations (CPAMs), congenital lobar or segmental emphysema and pulmonary sequestrations (PS) also form space-occupying lesions during fetal development and are often diagnosed prenatally. The exact pathophysiology of CPAM development remains controversial with some authors postulating that it arises from arrested development of localized portions of the bronchial tree during the sixth to seventh week of fetal development while others believe they are hamartomatous lesions of the bronchial tree.29 CPAMs are characterized as a multicystic lung mass resulting from a proliferation of terminal bronchiolar structures with associated suppression of alveolar growth.30 In contrast to CDH, in cases of CPAMs and other CLL, the lung grows and then is compressed by the mass. Consequently, alveolization and bronchial branching is largely preserved in the other areas of the lungs. As a result, following resection or regression there can be normal development of other areas of lung tissue and these patients do not tend to suffer the long-term consequence of prenatal mass effect seen in patients with CDH. The postnatal morbidity and mortality is usually related to the size of the mass.
CPAM and bronchopulmonary sequestration are the most common congenital lung malformations that usually cause no physiologic perturbation prenatally. The natural history and clinical spectrum of these anomalies are variable, but they appear to depend mostly on the size of the mass and the secondary physiologic derangement. The growth of CPAMs usually plateaus between 25 to 28 weeks at which time the fetus appears to grow around the lesion. Most small- to moderate-sized lesions represent 90% to 95% of CPAMs and remain asymptomatic during fetal life. In contrast, large lesions represent 5% to 10% of CPAMs and may produce significant mass effect, which can lead to pulmonary hypoplasia and associated pulmonary hypertension, impaired fetal swallowing and polyhydramnios, and impaired fetal circulation and heart failure. Congestive heart failure in the fetus, known as nonimmune fetal hydrops, is defined by the presence of skin or scalp edema, or by fluid accumulation in two or more serous cavities (ascites, or pericardial or pleural effusions). The risk of hydrops appears to depend on the size and rate of growth of the mass, and results from compression of the SVC and impaired venous return.31 In the past, hydrops was considered a harbinger of fetal demise, and was associated with near 100% mortality.32 Rarely, hydrops is due to a tension hydrothorax from extralobar sequestration. As a prognostic factor, the CCAM volume ratio (CVR) has been developed to correlate the relative size of these lesions with fetal and postnatal outcome.33 In one series in which 58 fetuses with a lung mass were followed prospectively, 75% of fetuses (12 of 16) with CVR greater than 1.6 developed hydrops, whereas only 17% (7 of 42) with CVR 1.6 or less had this complication. However, the CVR was not predictive of hydrops if a CCAM had a dominant cyst which may enlarge at an unpredictable rate. If signs of hydrops appear, the treatment options include percutaneous placement of a thoracoamniotic shunt for lesions with a dominant cyst, maternal steroids, and rarely open fetal thoracotomy and mass resection if the fetus does not respond to steroids. Although not well understood, results from a small series of patients suggest that maternal administration of betamethasone should be first-line therapy for all fetuses with a large lung mass (CVR >1.6), and for those with hydrops.34,35
Preterm Labor
For every fetal surgery, there is risk of preterm delivery. Chorioamniotic membrane separation and preterm labor remain the Achilles’ heel of fetal interventions.36,37 Surgical approaches that involve a smaller hysterotomy and more minimally invasive techniques appear to lower these risks.38 Specifically, fetoscopic and percutaneous approaches lessen the maternal and fetal risks. These techniques allow vaginal delivery after fetal surgery and appear to decrease the incidence of preterm delivery.
Preterm labor is the single biggest concern during the operation and in the postoperative period. The mother receives a 50-mg indomethacin suppository before surgery, and remains on indomethacin for 48 hours postoperatively. Daily echocardiography is essential; if there is evidence of PDA restriction, then the indomethacin is stopped. During the operation, nitroglycerin infusion can be used to help control uterine irritability and enhance relaxation. During closure of the hysterotomy, the mother is given a 6-g bolus dose of magnesium sulfate followed by a continuous infusion of 2 to 4 g per hour for up to 48 hours. Postoperatively the mother is closely monitored for pulmonary edema, fluid management, and uterine irritability. On the second to third postoperative day, usually the magnesium and indomethacin can be weaned, and the patient is converted to oral nifedipine.
Neonatal and Pediatric Physiology
Neonatal and Pediatric Lung Mechanics
The mechanical properties of the lung play an important role in pulmonary mechanics and neonatal respiratory physiology. The lung has physical properties which resist inflation including recoil, resistance, and inertance with the dynamic interaction between these processes being responsible for the effort required during normal spontaneous breathing.39 Elastic recoil is a property of the elastic lung tissue which must be stretched for lung inflation to occur. According to Hooke’s law, the pressure needed to inflate the lung must be proportion to the volume of inflation. This relationship of proportionality is change in volume divided by change in pressure or lung compliance. Dynamic compliance is the volume change divided by the peak inspiratory transthoracic pressure. Static compliance is volume change divided by peak inspiratory pressure.40 Throughout the range of normal tidal volumes, this relationship is linear and starts to plateau at large lung volumes. On a static compliance curve, ventilation normally occurs in the steep portion were large changes in volume occur for small changes in pressure. This is not true at high or low lung volumes where large changes in pressure are necessary for smaller changes in volume.
The total compliance for the pulmonary system is made up of the compliance of the lung and the chest wall. The relationship can be expressed by the equation: respiratory system compliance (CRS) = 1/chest wall compliance (CCW) + 1/lung compliance (CL).39 The desire for the lung to collapse is balanced by the outward elastic recoil of the chest wall with FRC occurring at the end of expiration when these forces are in equilibrium. In infants, the chest wall is composed primarily of cartilage and thus the CCW is greater in the infant and the pleural pressure is less negative. As a result of the more compliant chest wall, the neonatal lung appears more prone to collapse.41 FRC is maintained in newborns by increasing expiratory resistance through laryngeal abduction, maintaining inspiratory muscle activation throughout expiration, and initiating high breathing frequencies to limit expiration time.42
Resistance to gas flow is also an important determinant of pulmonary gas movement in the neonate. Resistance to airflow arises due to friction between gas molecules and the walls of the airway and because of friction between the tissues of the lung and the chest wall. The airway resistance makes up a significantly greater proportion of the resistance making up approximately 80% of the total resistance with 50% of the airway resistance.43 During laminar flow the pressure difference necessary for gas to flow through the airway is directly proportional to flow times a rate constant-airway resistance.39 During turbulent flow, which occurs at branch points, sites of obstruction, and high flow, this pressure necessary to move air is directly proportional to a constant times the flow rate squared. This constant is directly proportional to the volumetric flow rate and gas density and is inversely proportional to the radius of the airway and gas viscosity. Airway diameter is possibly the most important clinical factor effecting airway resistance because airway resistance varies inversely with the radius to the fourth or fifth power. Airway resistance is decreased during inspiration as the pleural pressure becomes negative due to expansion of the chest wall which increases airway and alveolar diameter and decreases resistance. During expiration, the pleural pressure increases and the airways are compressed. Collapse is prevented by cartilage and gas pressure in the lumen which can become a problem especially in small preterm infants with poorly supported central airways.
Work of breathing is the amount of energy required to overcome the elastic and resistive elements within the pulmonary system and move air in and out of the lungs. This is the cumulative product of distending pressure and the given volume displaced during inhalation or exhalation.39 In neonates, this is approximately 10% of the energy expenditure of an adult and the majority of work is done by the diaphragm. One-third of the inspiratory work is presumed to overcome the resistance of gas flow in the airways.
Evaluation of Lung Function
Knowing the terminology describing static lung measurement is essential to describing pulmonary function (Fig. 99-3). Assessing lung function in the neonates can be difficult as they are unable to cooperate with the examination. However, assessing and understanding lung function is essential to understanding the physiology, pathophysiology, and response to therapeutic intervention. Tidal volume, vital capacity, inspiratory capacity, inspiratory reserve volume, and expiratory reserve volume can be measured directly using spirometer. On the other hand, total lung volume, FRC, and residual volume require specialized techniques. Over the last 50 years, technologic advances have allowed for measurements of neonatal lung function to move from the bench top to the clinic with new ventilators able to provide breath-to-breath analysis of lung function.
The respiratory cycle is determined by changes in pressure which drives air in and out of the lungs. During normal inspiration, the respiratory muscles contract moving the diaphragm inferiorly and chest wall outward resulting in a transient decrease in the transpulmonary pressure from 0 to subatmospheric. This pressure gradient peaks during midinspiration with maximal airflow at that time. It subsequently returns to 0 at the end of inspiration. As the muscles of respiration relax, the inward recoil increases the transpulmonary pressure causing an expiration of inspired air. The airflow again reaches its maximum during midexpiration and returns to zero at the end of respiration. This results in a cycle of pressure within the alveoli from negative to positive pressure during normal inspiration.
Measuring these characteristics of ventilation including pressure, volume, and flows requires specialized equipment and methodologies. Over time, the devices to measure these parameters have continued to significantly improve. Pneumotachometers are one device which can be utilized to airflow. These instruments use gas flow through a tube containing a fixed laminar flow element or flow-resistive–type device. Gas flow across the element causes changes in pressure which can be measured by pressure transducers. An additional means of measuring airflow is the use of hot wire anemometers. These devices measure the amount of current which is required to keep a fine wire a constant temperature with current increasing as airflow increases.13,44 Volume measurements can be obtained using a flow sensor and by the integration of the flow signal over time. This is now performed digitally.
New methods to examine lung volumes have been investigated focusing on using techniques which do not require patient cooperation or need to connect to the open airway due to difficulty with leaks and the influence attempts at measurement can have on neonate breathing patterns. For example, one such technique which has been examined and validated in adults is optoelectronic plethysmography which estimates chest wall volume using several three-dimensional markers placed on thorax. This can accurately measure lung volume changes.45 In order to obtain meaningful static lung measurements FRC can be measured using whole body plethysmography, however, this is not a practical means for measuring FRC.46 Other methods for measuring FRC including helium dilution, nitrogen washout, and sulfur hexafluoride washout, have been utilized but can be challenging in an uncooperative, nonmechanically ventilated infant.
Figure 99-3. Lung measurements: Tidal volume (TV) is the amount of gas moved during one normal inspiration and expiration. Functional residual capacity (FRC) represents the volume of gas left in the lung following normal expiration. Inspiratory capacity is the maximum volume of air which can be inspired following a normal expiration. Inspiratory reserve volume is the additional amount of air which can be inspired following normal inspiration. Expiratory reserve volume is the additional amount of air which can be expired following normal expiration. Residual volume is the minimum lung volume possible, which is the air which remains in the lung following maximum expiration. Vital capacity is the maximum amount of air which can be moved, maximum inspiration following maximum expiration. Total lung capacity is the total amount of volume present in the lung.
Assisted Ventilation
While in most instances, transition from placental support to respiratory self-sufficiency occurs without complications, pediatric surgeons encounter many clinical scenarios where patients require additional assistance and mechanical support of the newborn has been a growing field with many advances. Neonatal respiratory failure has been treated with mechanical ventilation since the 1960s.47 Initially, adult ventilators were modified for neonatal use. The first devices designed for neonates were continuous flow, time cycled, pressure limited devices to provide intermittent mandatory ventilation (IMV).48 There were many limitations to these ventilators as physicians could only vary fraction of inspired oxygen, peak inspiratory pressure, positive end-expiratory pressure, inspiratory time, rate, and circuit flow. In the 1980s, high-frequency jet ventilation (HFJV) was introduced and has since been widely used. During the 1990s, microprocessors were incorporated into neonatal ventilators greatly expanding the scope of neonatal ventilation with clinicians able to control a greater number of variables including target modality, mode of ventilation, minute ventilation, cycling mechanism, assist sensitivity, and rise time with light weight transducers giving real time breath-to-breath information allowing easier adjustment of ventilator settings depending on patient illness.49
Currently, ventilators in clinical use are classified as either those which deliver tidal ventilation (conventional) or devices which deliver smaller gas volumes at rapid rates (high-frequency ventilators). Conventional ventilators have significantly improved over recent years with the addition of the microprocessor chips. They continue to have standard modes of ventilation including IMV, synchronized intermittent mandatory ventilation (SIMV), assist control ventilation (AC), and pressure support venation (PSV). These modalities can be further modified to deliver either target pressure or volume. Further description of conventional ventilators is beyond the scope of this chapter, however, the clinical utility of various modalities has been examined in recent literature. For example, a recent meta-analysis of pressure-controlled modalities versus volume-controlled modalities demonstrated a significant decrease in pneumothorax and duration of ventilation as well as a decrease in CLD in preterm infants treated with volume-controlled modes of ventilation as opposed to pressure-controlled modes.50
There are two types of high-frequency ventilation: HFJV and high-frequency oscillatory ventilation (HFOV). HFJV provides smaller volumes (1 to 3 mL/kg) more often at a much higher rate (240 to 660 breaths per minute) and expiration is passive. Oxygenation is proportional to mean airway pressure and ventilation is proportional to amplitude (peak inspiratory pressure vs. PEEP).48 Jet pulsations produce high-velocity laminar flow which has the ability to bypass airway disruptions. HVOF differs in that it delivers smaller tidal volumes (1 to 2 mL/kg) and at an even faster rate (8 to 15 Hz). The lung is inflated to a static volume, and then oscillated around the mean airway pressure.
Neonatal and Pediatric ECMO
For infants who cannot be successfully ventilated using these modes of ventilation, extracorporeal life support (ECLS) can become an option. ECLS is indicated in cases of acute cardiopulmonary failure with expected organ recovery which has been unresponsive to maximal therapy.51 The use of ECLS in neonates is based on the idea that with maximal support native function improves, through natural development or improvement, by application of medical and/or surgical treatments, or transplantation. Three clinical measurement systems have been identified to identify these patients all of which are associated with high mortality risk. However, the decision to initiate this therapy is based on clinical judgment and the patient’s response to maximal medical therapy.
Dr. Robert Bartlett and his group had been studying extracorporeal support in laboratory animals for 10 years before the first neonatal extracorporeal membrane oxygenation (ECMO) patient was supported in 1975; this clinical application signified a radical shift in the treatment paradigm for neonatal respiratory failure.52 The neonate, Esperanza, was suffering from respiratory failure secondary to meconium aspiration that was recalcitrant to conventional ventilator support. She was successfully supported and now is 37 years old.53
The currently accepted treatment of neonatal cardiopulmonary failure includes low-volume protective ventilation,54 inhaled nitric oxide (iNO),55,56 surfactant therapy,57,58 and HFOV.59 If the cardiac or pulmonary failure is refractory to maximal medical therapy then ECMO should be considered.60,61 The standard application of ECMO is primarily limited to neonates ≥34 weeks EGA and ≥2 kg. Clinical experience and laboratory work suggest extracorporeal support may be effective at lower gestational ages by using “Premie ECMO” or the “Artificial Placenta (AP) (Table 99-1).”
Indications
ECLS is indicated for acute cardiopulmonary failure with high mortality/morbidity unresponsive to maximal medical treatment and with expected organ recovery. The premise is that patients can be supported with ECLS while native function improves either by allowing natural development or improvement (lung maturation and surfactant development), application of medical or surgical therapies, or by transplantation. Identification of the patients that can benefit from ECLS can be challenging.
Three clinical measurement systems have been developed and tested to assist in identifying patients that will benefit from ECLS support.
1. Oxygenation Index (OI) = (MAP × FiO2 × 100) / PaO2 Where MAP = mean airway pressure. This index has been evaluated62,63 and shown that an OI >40 in three to five postductal gases was predictive of a mortality risk ≥80%.64
2. Postductal Alveolar-Arterial Oxygen Gradient [(A-a)DO2] An (A-a)DO2 of 610 Torr or greater despite 8 hours of maximal medical therapy predicted a mortality of 79%.63
3. Ventilation Index = (Respiratory Rate × PaCO2 × Peak Inspiratory Pressure)/1,000
Rivera et al. found that a ventilation index >40 and OI >40 were associated with a 77% mortality risk. They also found that the combination of peak inspiratory pressure ≥40 cm H2O and an (A-a)DO2 >580 mm Hg was associated with a mortality of 81%.65
These clinical measurement systems are useful to quantitate the degree of cardiopulmonary derangement, and subsequently categorize patients into candidates for ECLS therapy or continued maximal medical therapy. However, the decision to initiate ECLS therapy is often a clinical decision based upon clinical judgment and the patient’s individual response to maximal medical therapy. Patients are commonly started on ECLS therapy when they have failed maximal medical support, significant barotrauma is imminent, and are felt to have good potential for complete organ recovery.
Classic Contraindications and Possible Treatment Expansion
The classic contraindications to ECLS therapy are listed below. As ECLS treatment evolves and technology advances, many of the classic contraindications to ECLS are being challenged.
1. EGA less than 34 weeks: The higher incidence of intracranial bleeding in premature infants has historically precluded the use of ECLS in neonates less than 34 weeks EGA.66,67 However, recent data indicate that ECLS can potentially be used in infants as low as 29 weeks EGA with acceptable survival and intracranial hemorrhage (ICH) rates. Ideally, development of nonthrombogenic coating of circuit components would obviate the need for systemic heparinization and decrease the risk of using ECLS in premature infants.68–71
2. ICH greater than grade II: Neonates with ICH of higher grades are at increased risk of extension of their hemorrhage with systemic heparinization. This remains true today, but the development of technologies that obviate the need for heparinization may allow the use of ECLS in neonates with pre-existing ICH in the future.69,71–75 In addition, our experience has suggested that ECMO can be applied when expected mortality is higher in neonates with grade II ICH. In that setting, lower levels of anticoagulation are cautiously applied.
3. Mechanical ventilation for longer than 7 to 10 days: Classically, mechanical ventilation has been associated with higher incidence of bronchopulmonary dysplasia and irreversible fibroproliferative lung disease. The duration of pre-ECMO mechanical ventilation is being challenged; data from the Extracorporeal Life Support Organization (ELSO) registry demonstrate survival of 50% to 60% after pre-ECLS mechanical ventilation of up to 14 days.76
4. Cardiac arrest which requires cardiopulmonary resuscitation (CPR): Many centers now consider patients who suffer pre-ECLS cardiac arrest candidates for support. Survival rates up to 60% have been demonstrated in neonates who suffer cardiac arrest prior to or during cannulation.77,78 Predictably, good outcomes are associated with effective CPR during the resuscitation.
5. Conditions incompatible with meaningful life after therapy – profound neurologic impairment, congenital anomalies, or other conditions: With improvement in medical and surgical care, conditions once thought to be nonsurvivable require constant reassessment.
Outcomes
Today, ECMO is a part of routine management in the neonatal ICU. Overall survival is 85%, with 98% survival for meconium aspiration and 55% survival for diaphragmatic hernia.52 Overall survival after ECLS for neonatal respiratory failure has recently declined. There are likely a few reasons for this decrease in survival. Since its peak in 1992 of 1,500 cases, ECLS has been used with less frequency for critically ill neonates. The fewer number of cases is due to an improvement in other modalities of support such as iNO, HFOV, and surfactant therapy. To some extent, these improvements have been realized as a result of lessons learned from early ECMO experience.52,79–81 As a result, the neonatal patients receiving ECLS as the initial form of therapy for cardiopulmonary failure are declining, and the patients that ultimately require ECLS are probably more ill and further along the timeline of their illness.
Table 99-1 ECLS Modality by Age Group
Modalities
ECLS aims to provide perfusion of warmed, arterialized blood into the patient.82,83 Traditionally, venovenous (VV) support has been used for respiratory failure while venoarterial (VA) support has been utilized in cases of cardiac or combined cardiopulmonary failure. VV-ECLS can be performed through one-site or two-site cannulation. Traditionally, blood was drained through a cannula placed in the right internal jugular (IJ) vein and returned through the femoral vein, although this approach proved problematic in newborns with small femoral veins. More recently one-site or venovenous double-lumen (VVDL) cannulation has emerged as the preferred cannulation technique. In this mode, a single cannula is placed in the right IJ vein. Deoxygenated blood is drained from one port, pumped through the ECLS circuit where gas exchange occurs, and returned through a separate port on the same cannula into the right atrium. VA-ECLS provides complete cardiopulmonary support. Typically a drainage cannula is placed in the right IJ. Deoxygenated blood is pumped through the ECMO circuit and returned through a cannula in the right carotid artery. Historically, VA-ECLS has been used more than VV-ECLS in the support of neonates. However, data from the ELSO registry demonstrate that the use of VV-ECLS is increasing for neonatal cardiopulmonary failure.83
ECMO II
The first generation of ECMO support devices (known as ECMO I) was used from 1975 to 2008. However, problems with the oxygenator membrane, rotational pump, and cannulae limited expansion of the technology. Recently there has been substantial technologic improvement in ECMO system components and circuitry, defined as ECMO II.84
Modern hollow fiber oxygenators have several advantages over the original silicon membrane oxygenators.85–87 The hollow fiber design provides a much lower resistance across the oxygenator membrane, allowing for the use of centrifugal pumps in the ECMO circuit. The centrifugal pumps used now consist of a rotating impeller that spins on a small bearing or is magnetically suspended.88 Some of the newest pumps available provide pulsatile flow.89 Additionally, the advent of the double-lumen Avalon cannula (Avalon Elite, Avalon Laboratories, Rancho Dominguez, CA) allows the percutaneous placement of a single cannula for VV-ECMO support that both drains and reinfuses blood to allow for effective circuit flow.90,91 Further, there has been significant research effort put forth to improve the biocompatibility of circuit surface–blood interfaces.72,73 Finally, the simplification of operating the ECMO II system makes it such that a trained ICU nurse can manage the circuit. This decreases manpower utilization, as a single ECMO technician may now oversee several patients at once.92 These advancements provide translational development in the application and expansion of ECMO support for neonatal cardiopulmonary failure.
ECMO in Premature Neonates
Previous studies have shown poor survival with the use of ECMO in premature neonates. Bartlett’s early report of ECLS in 45 moribund neonates, revealed a 55% survival rate for neonates overall. Nine of these patients were less than 35 weeks EGA – two survived (22%). All six who died had ICH.93 A later report showed similarly worrisome survival for premature neonates less than 35 weeks EGA – 33% (3/15). One of these survived with an ICH; the other 12 patients with ICH died.63 A study examining ICH in patients on ECLS showed that all eight of the patients less than 35 weeks EGA had ICH and that six of these died immediately after termination of ECLS while two died within 1 year of age.66 Given these poor survival rates (22% to 33%) and the extremely high rate of ICH (75% to 100%), Cilley recommended that the use of ECLS be contraindicated in neonates of less than 35 weeks EGA. However, examination of the ELSO database between 1988 and 1991, found that survival had improved to 63% and ICH rates decreased to 37% for premature neonates born at 34 weeks EGA or younger.66,67
We recently performed a comprehensive analysis of the ELSO database from 1976 to 2008. This analysis showed no statistically significant difference in the risk of IVH in infants 30 to 33 weeks EGA (21%) when compared to those 34 weeks EGA (17%), p = 0.195.94 The overall survival rate of 54% and ICH rate of 18% for the entire cohort of neonates on ECLS at 34 weeks EGA or younger suggest that the incidence of both mortality and ICH have decreased over time, with a more dramatic reduction observed in ICH. Although the survival for the 29- to 33-week EGA group was significantly decreased (48%) when compared to those patients with EGA 34 weeks (58%, p = 0.011), one could argue that these rates are clinically acceptable. Patients born at younger EGA are expected to have a more difficult hospital course. These data suggest that ECLS in the modern era should be explored at EGA as low as 29 weeks with reasonable survival and acceptable rates of ICH.
Rationale for an Artificial Placenta – Applying Fetal Physiologic Principles to the Treatment of Extreme Prematurity. ECMO is increasingly effective for late preterm to term infants (34 to 40 weeks EGA), and it might be beneficial for some infants who are 29 to 34 weeks EGA. However, a different paradigm is required for ECLS in extremely low gestational age newborns (ELGANs, 22 to 28 weeks EGA). ELGANs are at the greatest risk for death and poor long-term outcomes.95–97 In particular, respiratory failure in premature neonates contributes to significant mortality and long-term disability. Conventional mechanical ventilation is often inadequate to provide gas exchange and can cause trauma to the under-developed lungs. Even more advanced strategies developed to minimize the trauma of conventional ventilation, including surfactant replacement therapy, nitric oxide inhalation, and HFOV, may at times be insufficient to support these most fragile infants.98,99 In theory, the most effective solution is to create an AP that maintains fetal circulation and obviates the need for mechanical ventilation and resultant devastating barotrauma by completely bypassing the lungs.
Definition of the Artificial Placenta
1. Maintenance of fetal circulation and the intrauterine environment
2. No mechanical ventilation
3. Simulated fetal breathing with fluid-filled lungs
4. A novel form of a pump-driven VV or arteriovenous (AV) ECLS/ECMO with outflow via the right jugular vein or umbilical artery, respectively, and inflow via the umbilical vein
A VV-ECLS AP offers several potential advantages over an AV-ECLS system. First, it eliminates the use of arterial vessels that are prone to spasm. Placing a cannula in the right atrium through the jugular vein allows for a passive drainage process that reduces potential negative pressure and cavitation in the circuit. Additionally, VV-ECLS uses the subject’s own venous system as a blood reservoir, instead of requiring an external blood reservoir. Another potential advantage relates to the drainage cannula itself. Although it is feasible to advance a large cannula (10 to 12 Fr) through the umbilical artery into the aorta in preterm sheep, this is not possible in premature human infants due to umbilical artery size and vessel anatomy. It would be very difficult to place even a 6-Fr cannula through the umbilical artery of a premature human baby. On the other hand, a larger cannula (6 to 8 Fr) can be inserted into the right IJ vein in premature infants to allow for adequate drainage. Finally, a VV-ECLS AP operates in parallel with the systemic circulation. Similar to traditional VV-ECMO circuits, the fetal heart would experience little increased resistance and afterload from the parallel circuit.83,100 Conversely, an AV-ECLS system with an oxygenator placed in series has the potential to increase stress on the fetal heart and contribute to cardiac failure.101
Clinical Prognostic Factors
The ideal patient population to benefit from the AP are ELGANs (<28 weeks EGA) who fail the most aggressive pulmonary therapies, such as low peak pressure ventilation, nitric oxide inhalation, surfactant administration, and HFOV. Early identification of those with the highest mortality risk has been validated in this population using mortality prediction tools (CRIB II and SNAPPE II) based on data obtained in the first 12 hours of life.102–104
Potential Complications and Current Limitations
There are several potential complications and questions that must be addressed in the laboratory before the AP can be taken to the clinical setting. The first, and most pressing, is the issue of anticoagulation and the risk of intracerebral or intraventricular hemorrhage (IVH). Issues with IVH were the main driving force for limiting conventional ECMO to late-gestation newborns.67,105 However, many of these reports were from a time before activated clotting time (ACT) was routinely followed in systematically anticoagulated neonates, leading to excessive anticoagulation. More recent studies on the pathogenesis of IVH suggest that many causes may be iatrogenic. The mechanism of injury may be either due to a disruption of cerebral blood flow or injury to the relatively weak endothelial tissue of the germinal matrix.106,107 Disruption of cerebral blood flow can be caused by transfusion or rapid volume expansion that leads to increases in arterial pressure and cerebral blood flow, or through positive pressure ventilation that may obstruct venous outflow and result in increased cerebral perfusion pressures.106,108 Avoiding ventilation and providing stable hemodynamics and gas exchange might afford the same protection against IVH. Nevertheless, to further minimize the risk of IVH, we propose the eventual use of nonthrombogenic surfaces in the form of biomaterials that release NO to completely eliminate the need for anticoagulation.69,71,74,75
Prematurity
Extremely premature infants are still “fetuses” living outside the womb and the physiology, pathophysiology, and treatment should be viewed through the lens of fetal physiology. Premature birth (<37 weeks EGA) occurs in 11% of births in the United States and remains the leading cause of morbidity and mortality in industrialized nations accounting for 60% to 80% of deaths in infants without congenital abnormalities.109 Prematurity affects most organ systems and includes temperature instability, respiratory failure and CLD, gastrointestinal problems including necrotizing enterocolitis (NEC), ICH, and sepsis. ICH occurs in approximately 12,000 premature infants every year with 50% to 75% of the survivors developing cerebral palsy, mental retardation, or hydrocephalus and another 25% of the nondisabled survivors developing psychiatric disorders or problems with executive function.110 Prevention, early recognition, and timely management are crucial in decreasing long-term morbidity and mortality.
The more premature the infant, the greater the risk for pulmonary complications with ELGANs born before 28 weeks EGA at greatest risk. As mentioned earlier, these infants are born at the transitional period between the canalicular and saccular phases which is when the air–blood barrier is just beginning to thin to the point where exchange is possible. Glucocorticoids are administered to mothers undergoing preterm labor after 24 weeks in order to promote surfactant production and maturation of the fetal lung.111,112 The exact mechanism remains unknown, however, studies have shown that anatomic maturation, in particular thinning of the alveolar wall, and synthesis of mRNAs encoding surfactant proteins increase with exposure and while with removal mRNAs decrease, anatomic changes persist.113–115
Operating on newborn infants, especially premature, can be technically challenging just by size alone. As the size of the infants can vary from a few hundred grams to several kilograms, surgeons must consider various technical and physiologic aspects of each procedure, choosing the safest and most effective option.
Several developmental and physiologic issues complicate surgery on premature infants. Lack of thermoregulation, inadequate skin integrity, and incomplete immune development are ubiquitous to all premature infants and can affect the morbidity of surgery. These aspects must be considered in all children, but most of all in the smallest patients, when choosing the location for surgery, transportation of the child, skin preparation, use of prophylactic antibiotics, placement of foreign bodies, postoperative wound management, and healing potential. Furthermore, specific physiologic processes inherent to the premature infant further complicate surgical planning.
Propensity for certain physiologic conditions in the neonate increases the risk of morbidity of operating on these children: hypoglycemia, apnea and respiratory distress, and neurologic development. Though patients are commonly kept nil per os (NPO) prior to surgery, to decrease the risk of aspiration with anesthesia, surgeons need to assess the risks and benefits of this approach for neonates who are at significant risk for hypoglycemia. As line access for neonates may tenuous and difficult to obtain, liberal use of enteral nutrition, appropriate timing of surgery, and early resumption of feeds in this population should be thoroughly considered. Furthermore, the use of narcotics and sedation can increase the risk of postoperative apnea and respiratory distress. The edema in the neonatal airway from intubation can contribute to early postoperative morbidity and need for reintubation or positive pressure ventilation; therefore, a conservative approach to extubation and postoperative monitoring should be used. Finally, although controversial, multiple studies suggest deleterious effects of anesthesia on the developing neonate and some recommend postponing elective procedures until children are 3 years of age.116–120
Thermoregulation
Fetal thermoneutrality is maintained in a warm intrauterine environment where the amniotic fluid reflects the maternal core temperature. In addition, the fetus generates 3 to 4 W/kg heat through an oxygen consumption of 8 mL/min/kg.121 The placenta eliminates 85% of fetal heat into the maternal circulation, the remaining 15% is dissipated through conductance to amniotic fluid and to the uterine wall. The fetus is at 0.5°C to 1.0°C above the maternal core temperature.
In neonates, thermoneutrality is defined as an axillary temperature between 36.7°C and 37.3°C.122 Soon after birth, approximately 135 to 155 W/m2 of heat loss can occur through evaporation, radiation, convection, and conduction.121 Therefore, a newborn exposed to cold stress must mount a variety of responses to conserve heat loss, increase heat production, and maintain core temperature. The main source of heat production is brown adipose tissue, which peaks at term gestation, used for nonshivering thermogenesis. Term newborns also increase involuntary activity, and have hypothalamic-regulated vasoconstriction to maintain thermoneutrality.13,121
Prematurity leads to insufficient brown fat for nonshivering thermogenesis, a larger surface area to body mass ratio, deficient subcutaneous fat and nonkeratinized thin skin, decreased ability to maintain flexion of extremities, and underdeveloped response of temperature sensors in the posterior hypothalamus to release thermogenic hormones (thyroxine and epinephrine).13 In low–birth-weight infants, the use of a servoregular set to 36°C has been shown to increase survival as compared to setting a constant incubator temperature. Radiant warmers increased insensible water loss and did no better when compared to incubators for temperature control.123
Neonates needing surgery are frequently transported to and from the neonatal ICU to the operating room and radiology, leading to increased risk of heat loss. Furthermore, since fever is an infrequent sign of postoperative infection, monitoring for temperature instability is more effective. Finally, perioperative issues such as intraoperative irrigation, exposure during positioning, skin preparation, and operating room temperature can all have ill effects on the susceptible neonate’s thermoregulation.
Skin Integrity
In addition to augmenting the risk of infant heat loss, poor skin integrity of neonates, especially the low birth weight or premature, has its own impact on the surgical patient. Beyond thermoregulation, the thin, underdeveloped skin has several other challenges including the use of local anesthetic, skin preparation, immune properties, bathing and wound care, and the use of an adhesive dressing. Skin provides mechanical protection, maintains thermoregulation, provides immunosurveillance, and prevents insensible loss of body fluids.124 Skin of premature infants is thinner and more dysfunctional. The inadequate epidermal barrier allows increased water loss, absorption of chemicals, and trauma causing difficulties with fluid homeostasis, thermoregulation, infection, and toxicity.125
Iatrogenic injury and alteration in skin integrity can occur more often in premature infants due to several factors. At 26 weeks, the developing epidermis is only 2 to 3 cells thick. At 28 weeks, the skin is deficient in fat and zinc, increasing the potential for infection, damage, and injury.126 Furthermore, due to the lack of protein in the subcutaneous layer, increased edema can form in the skin from excess water and sodium. The lack of sweat glands, a reduced blood supply, and lack of fat and calorie reserves, result in an increased risk of hypothermia.126
At birth, the baby’s skin is coated with vernix caseosa (a gel like substance that has antibacterial properties) in addition to blood, meconium, and cellular debris. The baby should not be bathed in the first 6 hours due to increased risk of hypothermia. Premature infants should have a bathing schedule approximately once every 4 days.124 In fact, bathing has been shown to reduce skin colonization, which may increase the risk of pathologic infection. Meticulous umbilical cord cleansing in the first 1 to 2 weeks, however, can markedly reduce infection and neonatal death. Emollients containing a physiologic balance of epidermal lipids – such as cholesterol, ceramide, palmitate, and linoleate – are optimum for barrier repair.127
Due to specific properties of neonatal skin, preoperative preparation should be carefully approached. Since neonatal skin is relatively impermeable to alcohol, it may develop skin burns and necrosis particularly in premature infants when topical antiseptic is used on skin.124 Therefore, alcohol-containing skin-cleansing solutions should be used with extreme caution in neonatal units. Iodinated skin disinfectants can result in significant iodine overload and severe transient hypothyroidism. Neonatal iodine exposure should be minimized whenever possible and in cases of exposure, thyroid-stimulating hormone level should be routinely measured.128 In general, we use betadine for most neonatal procedures, and chlorprep with hardware implantation (such as central line insertion), though no consensus currently exists.
Use of topical anesthetic similarly can cause systemic toxicity from absorption. Prilocaine, a component of topical anesthetics, can cause methemoglobinemia and cyanosis in neonates. Tetracaine gel can cause contact dermatitis.124 Furthermore, the use of lidocaine or marcaine in the subcutaneous space needs to be carefully monitored for overdose. We have used pumps that administer local anesthesia to the incision to limit systemic narcotic use in neonates with modest success.
Dressing the surgical wound in the neonate can create its own challenges. In premature infants, epidermal stripping secondary to tape and adhesive dressing removal is common and the primary cause of skin breakdown in babies in the neonatal intensive care units (NICU). On the other hand, adhesive removers should be avoided due to risk of skin injury and subsequent absorption.129 We routinely use steristrips as the sole dressing in children; alternatively skin glue can be an adequate dressing protecting the wound from friction and body fluids (urine, emesis, or feces). For open wounds, the use of a vacuum-assisted device (WoundVac) has been described and can be used selectively.130–132 For delicate skin, the use of Duoderm and Montgomery straps with wet-to-dry dressings is a good option.
Infection and Immune Response
Infection is the leading cause of mortality among infants in the first days of life,133 with mortality related to neonatal sepsis responsible for 40% of the annual 3 million worldwide neonatal deaths.134 Severe infections occur at a higher incidence and have greater mortality in very low–birth-weight premature neonates, especially in the first 3 to 7 days of life.135 Early-onset sepsis, associated with Escherichia coli or group B streptococcus (GBS), typically occurs within the first 24 hours, while late-onset sepsis, associated with nosocomial coagulase-negative Staphylococcus epidermidis, typically occurs after the first 72-hour period, and is most prevalent among very low–birth-weight babies.136 In neonates, central venous catheters are arguably the largest risk factor for neonatal nosocomial sepsis as coagulase-negative Staphylococcus and other organisms are the most commonly isolated late-onset pathogens, as they normally colonize the skin around the site or are acquired from the hands of the staff caring for the child.137
Maternal factors contributing to the risk of neonatal sepsis include prematurity, low birth weight, rectovaginal colonization with GBS, prolonged rupture of membranes, maternal intrapartum fever, and chorioamnionitis.135 Postnatal factors associated with an increased risk of sepsis include male gender, birth weight <1,000 g, hypogammaglobulinemia, intravenous alimentation, central venous catheters, the use of steroids or drugs that decrease gastric acid acidity, and prolonged duration of mechanical ventilation.135 Although the predominant agents are bacterial, viruses including herpes simplex and enteroviruses, can cause fatal neonatal sepsis.135 Invasive fungal infections can also contribute to mortality. Human milk, however, contains lactoferrin, an iron-binding glycoprotein that is important for innate immune host defenses at birth because it exhibits broad-spectrum antimicrobial activity and prevents invasive fungal infections.138 Neonates with sepsis may present in or progress to septic shock, exemplified initially by cardiovascular dysfunction requiring fluid resuscitation or inotropic support.139
Neonates, especially those that are premature, have an “immature” innate immune system, both quantitatively and qualitatively. For example, neonatal neutrophils have three- to fourfold less bactericidal permeability-increasing protein per cell than do adult neutrophils.140 The low immunologic profile of the newborn infant is possibly a reflection of the demands of the fetal environment, which is inherently considered sterile, and the need to avoid immune responses to maternal antigens.136 The development of neonatal immunity is influenced by multiple factors including “maternal cytokines, antigen exposure, and precursor frequency of lymphocytes and antigen presenting cells.”138
Neonates have dampened T-helper (Th) responses compared with adults. In fact, many murine and clinical studies have demonstrated decreased Th1-polarizing/proinflammatory responses (TNF-α, IFN-g, IL-12p70, IFN-α, IFN-γ), with increased production of Th2 polarizing (e.g., IL-6) and anti-inflammatory cytokine production (IL-10), following in vitro stimulation with bacterial products or septic challenge.136 This inherent bias toward Th2-cell polarizing cytokines and suboptimal Th1 responses and B-cell differentiation increases neonate susceptibility to acute respiratory and diarrheal diseases.138
Toll-like receptors (TLR), a type of pattern recognition receptors, exist on innate immune cells, leading to “second messenger-specific intracellular signaling cascadessic [sic] that result in gene expression, cytokine/chemokine production, and cellular activation.”136 Lipopolysaccharide (LPS, endotoxin) on gram-negative bacteria is a key mediator of systemic inflammation, septic shock, and multiorgan failure and death.141 TLR4, a receptor for LPS is critical for innate immune activation in leukocytes and is expressed on many epithelial cells. Neonatal leukocytes also demonstrate decreased responsiveness to LPS that signals through TLR4.136 LPS signals primarily through TLR4 in conjunction with the cell surface adaptor proteins CD14 and MD265.135 Cord blood monocytes exhibit higher expression of microRNA 146a (which regulates TLR4 signaling through the inhibition of IRAK4 – critical for downstream signaling) and appear to have higher endotoxin tolerance. Neonatal cord blood leukocytes also demonstrate reduced MyD88 expression and impaired LPS-induced p38 MAPK phosphorylation and LPS-induced proinflammatory cytokine production.136 These functional consequences of TLR activation in neonates are so markedly different from those in adults.
Figure 99-4. Zones of injury in ROP.
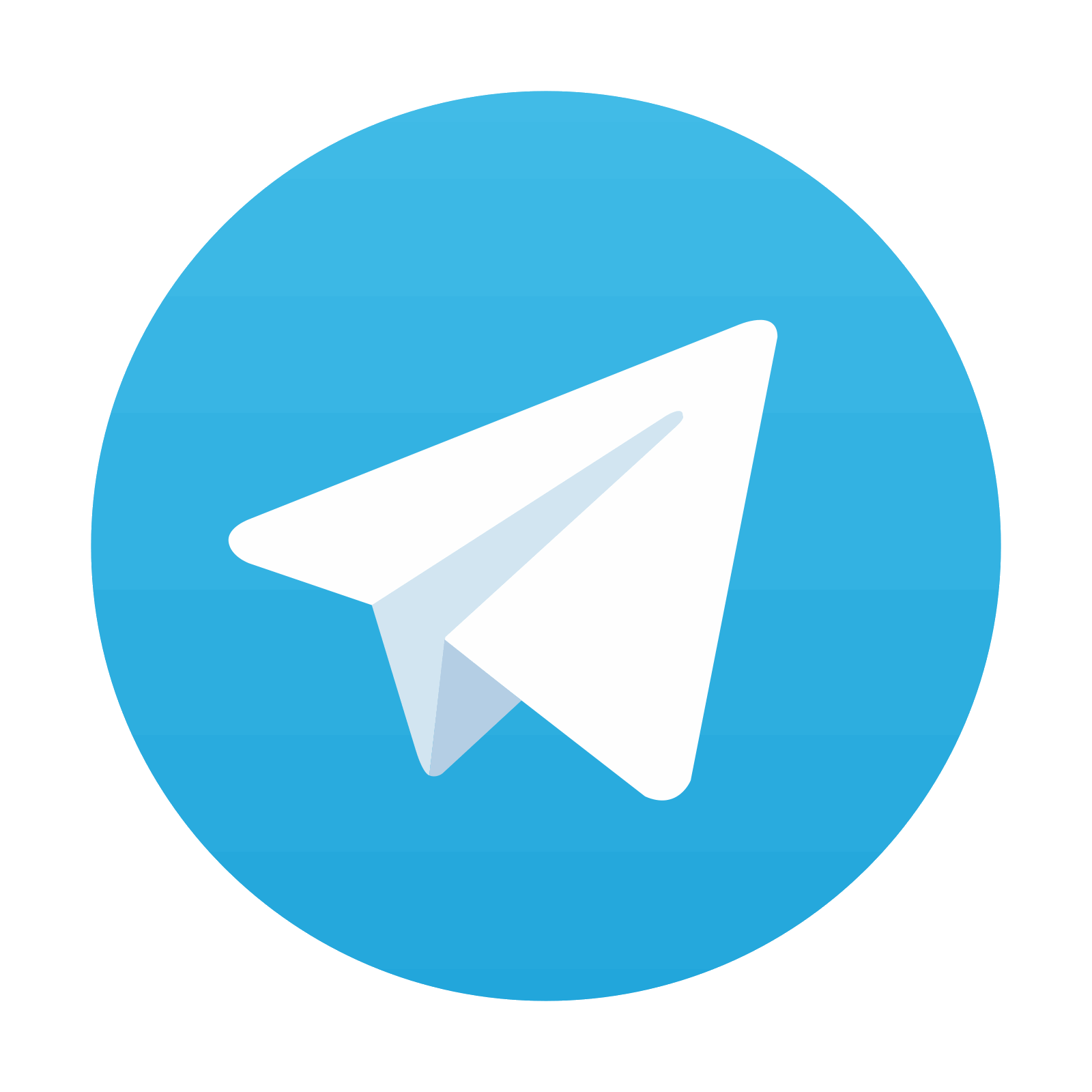
Stay updated, free articles. Join our Telegram channel
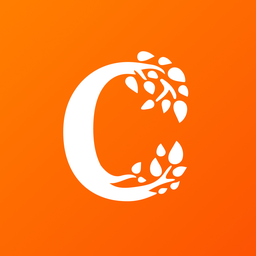
Full access? Get Clinical Tree
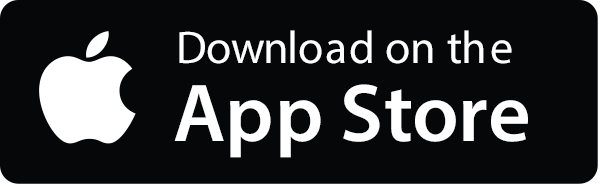
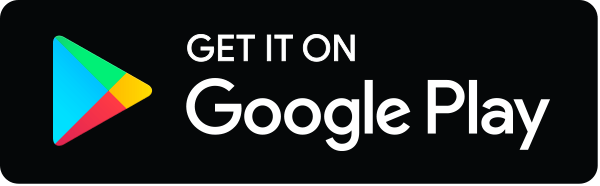