In this example, the large difference in magnitude between the time constants suggests that the two processes (i.e., the developmental transition in healing outcome and the healing process) occur at quite different rates, probably unfold separately, and can likely be studied largely independently of each other. In contrast, a healing process that hypothetically straddles across the early fetal–late fetal developmental transition in mammalian healing will probably reflect two or more different responses depending on whether the injury was inflicted just before, during, or just after the transition.
4.3 Three Modes of Defect Closure in Organs
Having experimentally isolated the healing process within a time segment, extending from injury (initial state) to wound closure (final state), we now wish to select a suitable method for studying its outcome. If the initial state is taken to be identical when investigators study anatomically well-defined defects, the configuration of the final state suffices to measure the effectiveness of a reactant to induce regeneration or fail to do so.
A survey of the literature of skin wound healing shows that no more than three processes (healing modes) are used to close a defect following injury: contraction of the dermal edges of the defect, formation of epithelialized scar, and regeneration (e.g., see review in Martinez-Hernandez 1988). The evidence shows that these three modes of closure are also responsible for closure of severe peripheral nerve defects, such as transection. Transected peripheral nerves close their stumps either by contraction of their diameter and formation of a neuroma (neural scar), or by regeneration (Chamberlain et al. 1998a; Soller et al. 2012). Additional, but much less extensive, evidence obtained with other organs of the adult anatomy, similar to that presented in Chap. 1, generally supports the conclusion that no mode of defect closure other than contraction, scar formation, and regeneration is observed throughout most of the anatomy.
4.3.1 Closure of Defects by Contraction
Contraction has been historically defined in extensive studies of skin wounds. Contraction of defects in other organs also has been observed in numerous studies but has not been studied systematically, as in skin.
Contraction of a skin defect is measured as the reduction in defect surface area by inward (centripetal) movement of skin from the margins of the defect (perilesional skin; Fig. 4.1). This process originates primarily with fibroblasts inside the defect and largely comprises the sliding, and secondarily stretching of perilesional skin over the open defect (Peacock and Van Winkle 1976; Rudolph et al. 1992; Troxel 1994). A detailed description of the mechanism of contraction during spontaneous healing is presented in Chap. 8. Even though it has been observed that the surface area of the skin defect decreases partly by a process such as sliding of perilesional skin (Troxel 1994; Gross et al. 1995), the term “contraction” has strong historical value and will be retained throughout this volume.

Fig. 4.1
Contraction kinetics of the dermis-free defect observed with three rodent models. Percent of initial defect area is plotted versus time of healing. Data for the guinea pig, rat, and mouse are shown. (From Kennedy and Cliff 1979)
Authors have generally assumed that the difference in extent of contraction of skin defects in different species reflects variation in skin mobility, itself largely the result of a difference in firmness of attachment of the skin to the subdermal tissues (tethering). For example, the skin of rodents has been described as a “fully mobile integument” (Billingham and Medawar 1951, 1955). Some authors have additionally emphasized the stiffness of the fascia that is attached to a major musculature underneath the skin (Peacock and Van Winkle 1976). In a sheep (Horne et al. 1992), rabbit, as well as in rodents (Billingham and Medawar 1951, 1955), the skin is attached relatively loosely to the panniculus carnosus, a layer of involuntary muscle underneath the dermis. In contrast, in the rabbit ear, the dermis is very firmly attached to a relatively rigid sheet of cartilage and is considered to be essentially immobile (Joseph and Dyson 1966; Goss and Grimes 1972).
The quantitative importance of contraction as a mode of skin defect closure has been established in kinetic studies with several species (Figs. 4.2 and 4.3). In species with mobile skin, such as the rabbit and several rodents, closure takes place almost entirely by movement of perilesional skin until its edges are opposed and separated by a thin layer of scar (stellate scar) (Billingham and Medawar 1951, 1955; Fig. 4.2). In sharp contrast, in the human and to a lesser extent in the swine, there is only modest movement of perilesional skin; full closure of the defect depends to a large extent on formation of an epithelialized scar layer over the newly synthesized connective tissue (often referred to as granulation tissue; Fig. 4.2, right; Peacock and Van Winkle 1976; Hayward and Robson 1991). In the rabbit ear, contraction is prevented and defect closure takes place entirely by regeneration (Joseph and Dyson 1966; Grimes and Goss 1972). Kinetic data show clear differences in the asymptotic extent to which contraction closes up a defect in different species, including the human (Fig. 4.3). The macroscopic mechanical force that suffices to close a skin wound in a rodent model has been estimated using a mechanical splint, at about 0.1 N (Higton and James 1964).

Fig. 4.2
Closure of dermis-free defects mostly by contraction (left) or partly by contraction (right). Left: Guinea pig. Right: Swine. Data in the two diagrams show the change in defect area viewed directly (en face) on the indicated day after excision. The defect area decreases much more rapidly in the guinea pig; it also approaches a lower asymptotic level, indicative of a larger extent of contraction and a smaller scar in this species. Arrows point to 10-day data. (Guinea pig data from Billingham and Reynolds 1952; swine data from Rudolph 1979)

Fig. 4.3
Kinetics of defect closure by contraction in three species, human (from Ramirez et al. 1969), swine (Rudolph 1979), and guinea pig (Yannas 1981). The percent of initial defect area that eventually closed by contraction was highest in the guinea pig and lowest in the human. The remainder of the defect area closed by formation of epithelialized scar
The detailed study of contraction of nerve stumps has seriously lagged behind similar studies in skin defects (Holmes and Young 1942; Weiss 1944; Weiss and Taylor 1944a; Sunderland 1990; Soller et al. 2012). Further information on contraction of transected peripheral nerves is provided below.
Defect contraction has been frequently reported following injury of several organs other than skin and peripheral nerves. Contraction has been reported as reduction in length of a slender organ (shortening), the constriction of a hollow organ (stricture), or the warping of a thin sheet-like organ. Examples of organ deformation following trauma in experimental animal models are the observation of contraction of the ureter following resection of a portion of the circumference (Oppenheimer and Hinman 1955; Kiviat et al. 1973) and the contraction of the transected ligament (Dahners et al. 1986; Wilson and Dahners 1988). In humans, the narrowing (stricture) of the urethra that follows trauma (Rudolph et al. 1992; Wong et al. 2012; Lee and Kim 2013); narrowing of the esophagus (esophageal stricture) after swallowing a corrosive agent (Peacock 1984) or after surgery (Singhal et al. 2013; Wu et al. 2013); and contraction of a defect in the upper eyelid (ectropion) after radiotherapy (Tarallo et al. 2012), have been usually attributed to the same contractile process that leads to closure of skin defects. Biologically inert implants, inserted inside an organ in a space that has been prepared by tissue excision, are typically covered by a capsule of contractile tissue; examples are silicone breast implants and cardiac pacemakers (Rudolph et al. 1992). The detailed structure of the contractile capsule surrounding breast implants has been described in great detail (Rudolph et al. 1978; Ginsbach et al. 1979; Brodsky and Ramshaw 1994; Tarpila et al. 1997; Hwang et al. 2010) and methods have been developed to measure the mechanical stiffness of the contracted capsule (Rzymski et al. 2011). Myofibroblasts were detected on the outer layer of the contracted capsule and made up about 27 % of capsule thickness while the tensile strength of the capsule was measured at 44 ± 38 N (Hwang et al. 2010).
Remarkably, contraction and scar formation both in the healthy neonatal and adult liver following a through and through incision have also been observed (Masuzaki et al. 2013). The cylindrical wound was generated by perforating the liver with a modified biopsy punch. A full-thickness 3-mm diameter injury was made with the biopsy punch on the left lobe of adult mice, 8 weeks of age. Following perforation, the sutures were placed in three locations around the perforated site, forming the apices of a triangle (Fig. 4.4; see points E, F, G in Fig. 4.4), and were photographed at the time of injury as well as 7 days after injury. During the 7-day period following the injury, the area of the triangle formed by the sutures was reduced considerably. A measure of the strain induced in the tissues in 7 days is indicated by the contraction of triangle side EF down to E′F′, i.e., a contraction to about 50 % of the original length (Fig. 4.4, Ho et al. 2011). Clearly, the perforated liver contracted substantially around the injured site.

Fig. 4.4
Wound contraction in the healthy, acutely injured liver (both neonate and adult) was measured following a cylindrical type of injury. The cylindrical wound was generated by perforating the liver with a modified biopsy punch. A full-thickness 3-mm diameter injury was made with the biopsy punch on the left lobe of adult mice, 8 weeks of age. Following perforation, sutures were placed in three locations around the perforated site, forming the apices of a triangle (see points E, F, G), and were photographed at the time of injury as well as 7 days after injury. During the 7-day period following the injury the area of the triangle formed by the sutures was reduced considerably, illustrating wound contraction. A measure of the strain induced in the tissues in 7 days is indicated by the contraction of triangle side EF down to E′F′, i.e., a contraction to about 50 % of the original length (Ho et al. 2011). Clearly the perforated liver contracted substantially around the injured site
Contractile fibroblasts (myofibroblasts) are commonly credited as the engine of wound contraction (Gabbiani et al. 1971; Rudolph 1979; Gabbiani et al. 1998; Hinz et al. 2004; Daimon at al. 2013). Characteristics of myofibroblasts and a discussion of the role they play in repair and regeneration are discussed in Chap. 8. Here, we simply show views of myofibroblasts in skin and peripheral nerve wounds. In an excisional full-thickness skin wound (Fig 4.5), contractile cells are seen to emerge in large numbers from the wound edges toward the center. In peripheral nerve wounds produced by transection, contractile cells surround the stumps (Fig. 4.6).

Fig. 4.5
Contractile cells extend across the entire area of the dermis-free defect in the guinea pig on day 9 post injury. Photo shows the leftmost edge of the skin defect only (edge) as it intersects the base (B). Bar: 200 µm. (From Troxel 1994)

Fig. 4.6
Contractile cells are abundantly present in the region of transection of a rat sciatic nerve at 11 day post injury. They appear as bundles of long fibers oriented along the axis of the regenerating nerve and appear to encapsulate the nerve perimeter. The photos show segments of a nerve trunk regenerated across a 10-mm gap in the rat sciatic nerve that was bridged with a silicone tube. The diameter of the intact nerve was about 1 mm. Top: Near location of original proximal stump. Bottom: Near original distal stump. (Chamberlain et al. unpublished data, 2000)
Traumatic injury is not the only cause of organ contraction; a chronic inflammatory process can also induce this process. Among the several examples of chronic organ contraction in humans are the narrowing and sometimes complete obstruction of the duodenum following repeated ulceration (Billingham and Russell 1956) and the shrinking of heart valves in rheumatic heart disease (Peacock 1984; Rudolph et al. 1992). A striking decrease in liver size, attributed to contraction, is a feature of terminal cases of liver cirrhosis (Rudolph et al. 1979a; Rudolph 1980; Nimni 1983) and has been attributed to contractile cell (myofibroblast) activity (Maruyama et al. 2011).
4.3.2 Measurement of Contraction in Skin and Nerve Defects
Quantitative studies of contraction of skin defects owe much to the methods devised by Spallanzani (Carrel and Hartmann 1916). In these early studies the methods of tattooing spots at the edges of the defect with India ink and of planimetry to measure defect area, as well as the mathematical modeling of the kinetics of contraction, had already been in place. Important changes in measurement technique made in later studies have included the systematic practice of measuring the defect area lying between the dermal edges rather than the edge of advancing epithelium (Billingham and Medawar 1955), the use of tattooed grids (rather than isolated spots) around contracting defects (Straile 1959; Alvarez et al. 1987) in order to locate the original defect perimeter without ambiguity, the use of computerized morphometric image analysis of standardized photographic slides of the defect (Staiano-Coico et al. 1993) and the use of near infrared spectroscopy to measure wound size by detecting hemoglobin concentration (Neidrauer et al. 2010).
There is evidence, obtained with the rabbit, rat, and guinea pig models, that defect size and the sex of the animal have no effect on contraction kinetics (Carrel and Hartmann 1916; Billingham and Russell 1956; Peacock and Van Winkle 1976; Kennedy and Cliff 1979). The assumption of independence of contraction from defect size should, however, be used with caution. It has been mentioned above (Chaps. 1 and 2) that defect size controls the incidence of scar versus regeneration in fetal skin defects (Cass et al. 1997b; Lovvorn et al. 1998). Furthermore, a defect large enough to extend to a quite different anatomical location would be expected to show a substantially different rate, and possibly a different major direction, of contraction. For example, in a mid-dorsal full-thickness skin defect in the rat, the lateral edges moved closer together than the anterior and posterior edges; in contrast, in a flank wound, the anterior and posterior edges moved closer than the lateral edges, suggesting that the contraction pattern was not uniform over the entire anatomy (Kennedy and Cliff 1979). Controversial data have been obtained relating the effect of wound shape on contraction rate. Although there is early evidence that circular wounds contract slower than rectangular wounds in the rabbit (Billingham and Russell 1956) and in the rat (Cuthbertson 1959), a later study showed no significant difference between the kinetics of contraction of rectangular and of circular wounds in the rat (Kennedy and Cliff 1979).
Measurements of the extent of skin defect closure by contraction have depended on direct observation of the surface of the defect rather than of tissues underneath. This description omits reference to the total mass of scar formed inside the volume of the defect that consists of two parts, visible and underlying scar. In the process of contraction, skin slides over newly synthesized connective tissue (granulation tissue) inside the defect. When the contraction process has stopped, the sliding skin has covered the underlying mass of granulation tissue, which eventually becomes modified to underlying scar. Histological observations on rodents have revealed the presence of a significant volume of scar, lying underneath the mobile contracted integument and presenting itself at the surface of the defect only as a thin stellate scar (Fig. 4.2, left; Luccioli et al. 1964; Peacock and Van Winkle 1976; Horne et al. 1992; Troxel 1994).
A transected peripheral nerve spontaneously heals with contraction and neuroma formation provided that the stumps have been separated by a few millimeters (mm) very soon after transection. Early reports of spontaneous contraction of the cross-sectional area of peripheral nerve stumps by as much as 50–60 % following transection were based on visual observation (Holmes and Young 1942; Weiss 1944; Weiss and Taylor 1944a; Sunderland 1990). When the nerve was tubulated, the extent of contraction changed dramatically. Although use of tubes fabricated from silicone elastomer led to contraction to about 47 % of the original cross-sectional area, much lower values of extent of contraction, close to zero, were observed when collagen tubes with specific structure were used (Chamberlain et al. 2000a; Soller et al. 2012).
Measurement of wound contraction in different organs has generally lagged seriously behind measurement of biochemical parameters of wound healing. A result of such paucity of data is typically a neglect of contraction in discussions of repair and regeneration. The critical importance of wound contraction in humans is illustrated in Fig. 4.7.

Fig. 4.7
Examples of skin wounds in humans that closed by contraction and scar formation. a Forearm of young boy following burn (From Tomasek et al. 2002). b Contraction and scar formation in burned chest and neck area in an adult female (Courtesy of Massachusetts General Hospital, Boston, MA). c Left: The burned foot of 15-year-old woman contracted and after 8 years without treatment developed into scar and a fixed deformity (contracture) in the area of the burn, which caused her little toe to be fixed in extension. Right: The scar was released through a series of surgical procedures (Z-plasties) that lengthened the scar and removed the tension. This allowed her toe to be placed in a normal position. (Photo courtesy of Dennis P. Orgill, M.D., Ph.D.)
4.3.3 Wound Closure by Epithelialized Scar in Skin and by Neuroma in Peripheral Nerve
In the adult mammal, the injured dermis does not regenerate spontaneously; scar forms instead (Billingham and Medawar 1955; Ross and Benditt 1961; Dunphy and Van Winkle 1968; Ross and Odland 1968; Peacock 1971, 1984; Madden 1972; Boykin and Molnar 1992; Goss 1992; Heng 2011). Provided that the depth of the injury extends well into the dermis, a layer of granulation tissue forms over the entire defect area (as well as underneath the advancing skin edges, as described above). Eventually, the defect area that has not been closed by contraction becomes epithelialized by migration of keratinocyte from the edges toward the interior of the defect and over the granulation tissue layer. The result is closure of the skin defect by epithelialized scar. In contrast with rodents, where contraction provides almost all of the closure of the wound, in the human as much as roughly one-half of the original defect area closes by formation of epithelialized scar. Skin appendages, such as hair follicles , sweat glands, and sebaceous glands , also do not spontaneously regenerate in the adult (Martin 1997).
Scar is not dermis in a variety of ways. The quantitative distinction between the two can, in principle, be made by several methods. In mechanical tests, scar is both less extensible than dermis and fractures at a lower-tensile stress (Dunn et al. 1985). A study using atomic force microscopy showed that scar tissue exhibited less viscoelastic creep and capability to dissipate mechanical energy than the upper dermis of intact skin (Grant et al. 2012). Morphologically, the normal dermoepidermal junction in scar appears abnormally smooth, typically lacking the complex rete ridge configuration of normal skin (Fig. 2.2, Kiistala 1972). The connective tissue layer of scar contains neither hair follicles nor sebaceous or sweat glands. Optical microscopic views of scar typically show collagen fibers preferentially aligned in the plane of the epidermis, packed more tightly and possessing an average diameter that is smaller than that of intact dermis. Scanning electron microscopic views have confirmed the high orientation of collagen fibers in scar (Hunter and Finlay 1976; Knapp et al. 1977; Ferdman and Yannas 1993). These morphological differences between normal dermis and scar were in agreement with changes after stretching observed by confocal laser scanning microscopy and other methods (Verhaegen et al. 2012). In contrast to scar, the dermis has been described as a sheet of collagen fibers aligned almost randomly; however, there is evidence of a modest amount of orientation of the axes of dermal collagen fibers in the plane of the epidermis (Gibson et al. 1965; Dawber and Shuster 1971; Brown 1972; Holbrook et al. 1982; Ferdman and Yannas 1993). Differences in chemical composition between normal dermis and scar also have been reported (Bailey et al. 1975; Matsumoto et al. 2002). No difference was observed between the axial periodicity of collagen fibrils, D = 65.5 ± 0.15 nm, in a granuloma (i.e., essentially scar tissue forming around an implant) and the value of D in normal skin (Brodsky and Ramshaw 1994). Measurement of the near-infrared attenuation coefficient using optical coherence tomography of burn scar tissue showed that scar had a weaker coefficient than normal skin, suggesting use of this optical method to assess human burn scars (Gong et al. 2014).
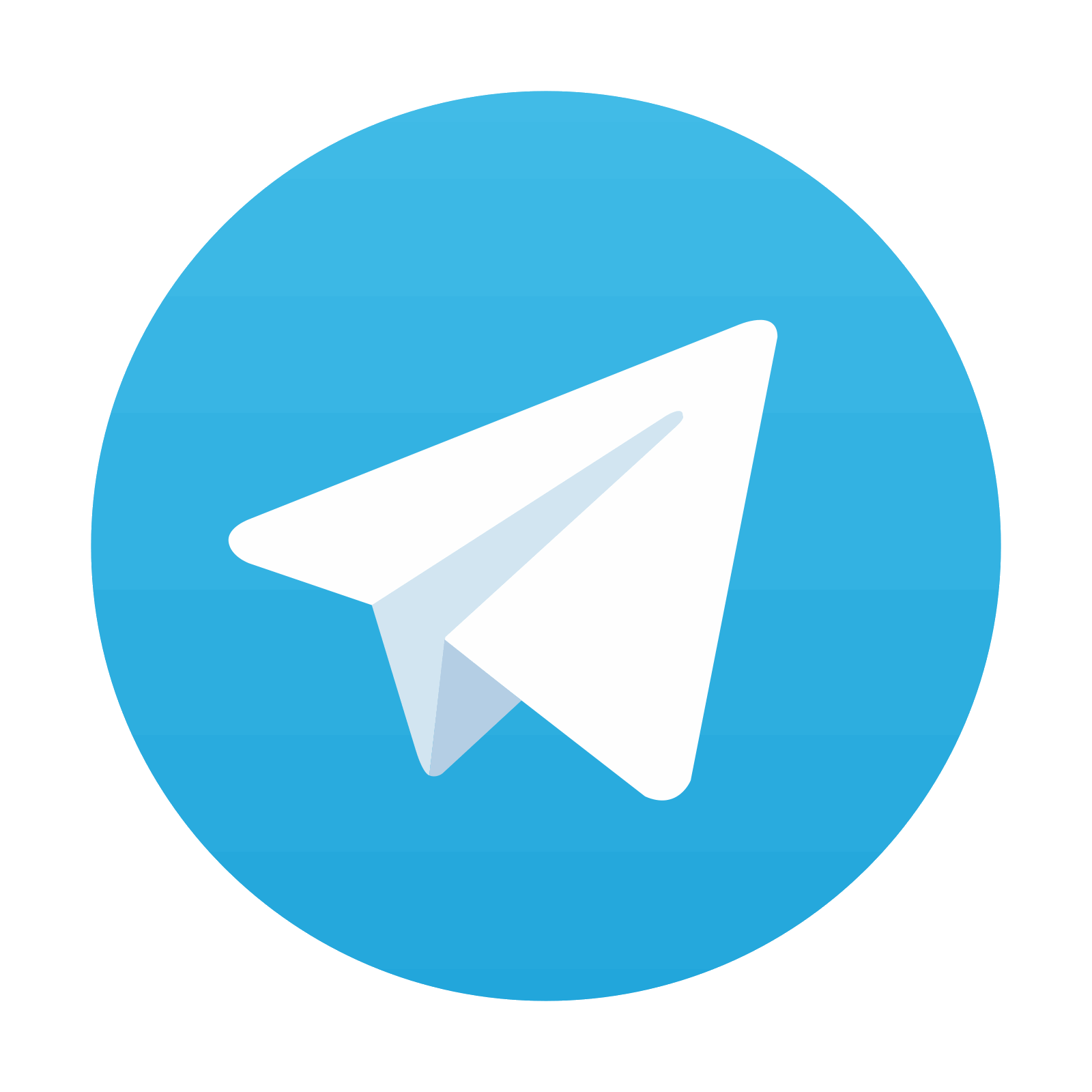
Stay updated, free articles. Join our Telegram channel
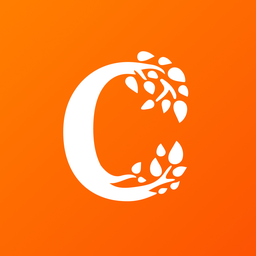
Full access? Get Clinical Tree
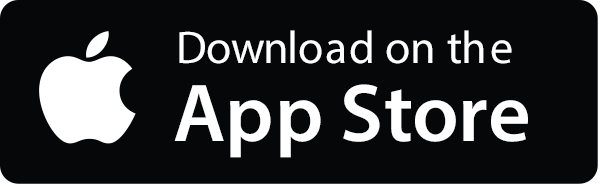
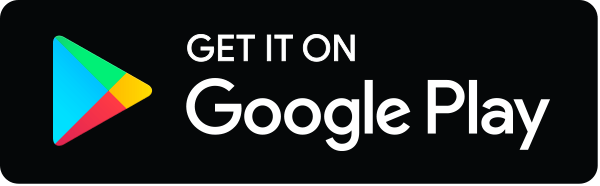