9
Enzymes: Regulation of Activities
Peter J. Kennelly, PhD & Victor W. Rodwell, PhD
OBJECTIVES
After studying this chapter, you should be able to:
Explain the concept of whole-body homeostasis and its response to fluctuations in the external environment.
Discuss why the cellular concentrations of substrates for most enzymes tend to be close to Km.
List multiple mechanisms by which active control of metabolite flux is achieved.
Describe the advantages of certain enzymes being elaborated as proenzymes.
Illustrate the physiologic events that trigger the conversion of a proenzyme to the corresponding active enzyme.
Describe typical structural changes that accompany conversion of a proenzyme to the active enzyme.
Describe the basic features of a typical binding site for metabolites and second messengers that regulate catalytic activity of certain enzymes.
Indicate two general ways in which an allosteric effector can modify catalytic activity.
Outline the roles of protein kinases, protein phosphatases, and of regulatory and hormonal and second messengers in initiating a metabolic process.
BIOMEDICAL IMPORTANCE
The nineteenth-century physiologist Claude Bernard enunciated the conceptual basis for metabolic regulation. He observed that living organisms respond in ways that are both quantitatively and temporally appropriate to permit them to survive the multiple challenges posed by changes in their external and internal environments. Walter Cannon subsequently coined the term “homeostasis” to describe the ability of animals to maintain a constant intracellular environment despite changes in their external environment. We now know that organisms respond to changes in their external and internal environment by balanced, coordinated adjustments in the rates of specific metabolic reactions. Perturbations of the sensor-response machinery responsible for maintaining homeostatic balance can be deleterious to human health. Cancer, diabetes, cystic fibrosis, and Alzheimer’s disease, for example, are all characterized by regulatory dysfunctions triggered by pathogenic agents or genetic mutations. Many oncogenic viruses elaborate protein-tyrosine kinases that modify the regulatory events that control patterns of gene expression, contributing to the initiation and progression of cancer. The toxin from Vibrio cholerae, the causative agent of cholera, disables sensor-response pathways in intestinal epithelial cells by ADP-ribosylating the GTP-binding proteins (G-proteins) that link cell surface receptors to adenylyl cyclase. The consequent activation of the cyclase leads to the unrestricted flow of water into the intestines, resulting in massive diarrhea and dehydration. Yersinia pestis, the causative agent of plague, elaborates a protein-tyrosine phosphatase that hydrolyzes phosphoryl groups on key cytoskeletal proteins. Dysfunctions in the proteolytic systems responsible for the degradation of defective or abnormal proteins are believed to play a role in neurodegenerative diseases such as Alzheimer and Parkinson’s. In addition to their immediate function as regulators of enzyme activity, protein degradation, etc, covalent modifications such as phosphorylation, acetylation, and ubiquitination provide a protein-based code for the storage and hereditary transmission of information (Chapter 35). Such DNA-independent information systems are referred to as epigenetic. Knowledge of factors that control the rates of enzyme-catalyzed reactions thus is essential to an understanding of the molecular basis of disease and its transmission. This chapter outlines the patterns by which metabolic processes are controlled, and provides illustrative examples. Subsequent chapters provide additional examples.
REGULATION OF METABOLITE FLOW CAN BE ACTIVE OR PASSIVE
Enzymes that operate at their maximal rate cannot respond to increases in substrate concentration, and can respond only to precipitous decreases in substrate concentration. The Km values for most enzymes, therefore, tend to be close to the average intracellular concentration of their substrates, so that changes in substrate concentration generate corresponding changes in the metabolite flux (Figure 9–1). Responses to changes in substrate level represent an important but passive means for coordinating metabolite flow and maintaining homeostasis in quiescent cells. However, they offer a limited scope for responding to changes in environmental variables. The mechanisms that regulate enzyme efficiency in an active manner in response to internal and external signals are discussed below.
FIGURE 9–1 Differential response of the rate of an enzyme-catalyzed reaction, ΔV, to the same incremental change in substrate concentration at a substrate concentration close to Km (ΔVA) or far above Km (ΔVB).
Metabolite Flow Tends to Be Unidirectional
Despite the existence of short-term oscillations in metabolite concentrations and enzyme levels, living cells exist in a dynamic steady state in which the mean concentrations of metabolic intermediates remain relatively constant over time. While all chemical reactions are to some extent reversible, in living cells the reaction products serve as substrates for—and are removed by—other enzyme-catalyzed reactions (Figure 9–2). Many nominally reversible reactions thus occur unidirectionally. This succession of coupled metabolic reactions is accompanied by an overall change in free energy that favors unidirectional metabolite flow (Chapter 11). The unidirectional flow of metabolites through a pathway with a large overall negative change in free energy is analogous to the flow of water through a pipe in which one end is lower than the other. Bends or kinks in the pipe simulate individual enzyme-catalyzed steps with a small negative or positive change in free energy. Flow of water through the pipe nevertheless remains unidirectional due to the overall change in height, which corresponds to the overall change in free energy in a pathway (Figure 9–3).
FIGURE 9–2 An idealized cell in steady state. Note that metabolite flow is unidirectional.
FIGURE 9–3 Hydrostatic analogy for a pathway with a rate-limiting step (A) and a step with a ΔG value near 0 (B).
COMPARTMENTATION ENSURES METABOLIC EFFICIENCY & SIMPLIFIES REGULATION
In eukaryotes, anabolic and catabolic pathways that inter-convert common products may take place in specific subcellular compartments. For example, many of the enzymes that degrade proteins and polysaccharides reside inside organelles called lysosomes. Similarly, fatty acid biosynthesis occurs in the cytosol, whereas fatty acid oxidation takes place within mitochondria (Chapters 22 and 23). Segregation of certain metabolic pathways within specialized cell types provides a further means for physical compartmentation.
Fortunately, many apparently antagonistic pathways can coexist in the absence of physical barriers, provided that thermodynamics dictates that each proceeds with the formation of one or more unique intermediates. For any reaction or series of reactions, the change in free energy that takes place when metabolite flow proceeds in the “forward” direction is equal in magnitude but opposite in sign from that required to proceed in the reverse direction. Some enzymes within these pathways catalyze reactions, such as isomerizations, that can act as bidirectional catalysts in vivo because the difference in free energy between substrates and products is close to zero. However, they represent the exception rather than the rule. Virtually all metabolic pathways proceed via one or more steps for which ΔG is significant. For example glycolysis, the breakdown of glucose to form two molecules of pyruvate, has a favorable overall ΔG of –96 kJ/mol, a value much too large to simply operate in “reverse” when wishing to convert excess pyruvate to glucose. Consequently, gluconeogenesis proceeds via a pathway in which the three most energetically disfavored steps from glycolysis are replaced by new reactions catalyzed by distinct enzymes (Chapter 20).
The ability of enzymes to discriminate between the structurally similar coenzymes NAD+ and NADP+ also results in a form of compartmentation. The reduced forms of both coenzymes are not readily distinguishable. However, the reactions that generate and later consume electrons that are destined for ATP generation are segregated in NADH, away from those used in the reductive steps of many biosynthetic pathways, which are carried by NADPH.
Controlling an Enzyme That Catalyzes a Rate-Limiting Reaction Regulates an Entire Metabolic Pathway
While the flux of metabolites through metabolic pathways involves catalysis by numerous enzymes, active control of homeostasis is achieved by the regulation of only a select subset of these enzymes. The ideal enzyme for regulatory intervention is one whose quantity or catalytic efficiency dictates that the reaction it catalyzes is slow relative to all others in the pathway. Decreasing the catalytic efficiency or the quantity of the catalyst responsible for the “bottleneck” or rate-limiting reaction immediately reduces metabolite flux through the entire pathway. Conversely, an increase in either its quantity or catalytic efficiency enhances flux through the pathway as a whole. For example, acetyl-CoA carboxylase catalyzes the synthesis of malonyl-CoA, the first committed reaction of fatty acid biosynthesis (Chapter 23). When synthesis of malonyl-CoA is inhibited, subsequent reactions of fatty acid synthesis cease for lack of substrates. As natural “governors” of metabolic flux, the enzymes that catalyze rate-limiting steps also constitute efficient targets for regulatory intervention by drugs. For example, “statin” drugs curtail synthesis of cholesterol by inhibiting HMG-CoA reductase, which catalyzes the rate-limiting reaction of cholesterogenesis.
REGULATION OF ENZYME QUANTITY
The catalytic capacity of the rate-limiting reaction in a metabolic pathway is the product of the concentration of enzyme molecules and their intrinsic catalytic efficiency. It therefore follows that catalytic capacity can be influenced both by changing the quantity of enzyme present and by altering its intrinsic catalytic efficiency.
Proteins Are Continuously Synthesized and Degraded
By measuring the rates of incorporation of 15N-labeled amino acids into protein and the rates of loss of 15N from protein, Schoenheimer deduced that body proteins are in a state of “dynamic equilibrium” in which they are continuously synthesized and degraded—a process referred to as protein turnover. This holds even for those proteins that are present at an essentially constant, or constitutive, steady-state level over time. On the other hand, the concentrations of many enzymes are influenced by a wide range of physiologic, hormonal, or dietary factors.
The absolute quantity of an enzyme reflects the net balance between its rate of synthesis and its rate of degradation. In human subjects, alterations in the levels of specific enzymes can be effected by a change in the rate constant for the overall processes of synthesis (ks), degradation (kdeg), or both.
Control of Enzyme Synthesis
The synthesis of certain enzymes depends upon the presence of inducers, typically substrates or structurally related compounds that stimulate the transcription of the gene that encodes them (Chapters 36 and 37). Escherichia coli grown on glucose will, for example, only catabolize lactose after addition of a β-galactoside, an inducer that triggers synthesis of a β-galactosidase and a galactoside permease (Figure 38–3). Inducible enzymes of humans include tryptophan pyrrolase, threonine dehydratase, tyrosine-α-ketoglutarate aminotransferase, enzymes of the urea cycle, HMG-CoA reductase, and cytochrome P450. Conversely, an excess of a metabolite may curtail synthesis of its cognate enzyme via repression. Both induction and repression involve cis elements, specific DNA sequences located upstream of regulated genes, and trans-acting regulatory proteins. The molecular mechanisms of induction and repression are discussed in Chapter 38. The synthesis of other enzymes can be stimulated by the interaction of hormones and other extracellular signals with specific cell-surface receptors. Detailed information on the control of protein synthesis in response to hormonal stimuli can be found in Chapter 42.
Control of Enzyme Degradation
In animals many proteins are degraded by the ubiquitin-proteasome pathway, the discovery of which earned Aaron Ciechanover, Avram Hershko, and Irwin Rose a Nobel Prize. Degradation takes place in the 26S proteasome, a large
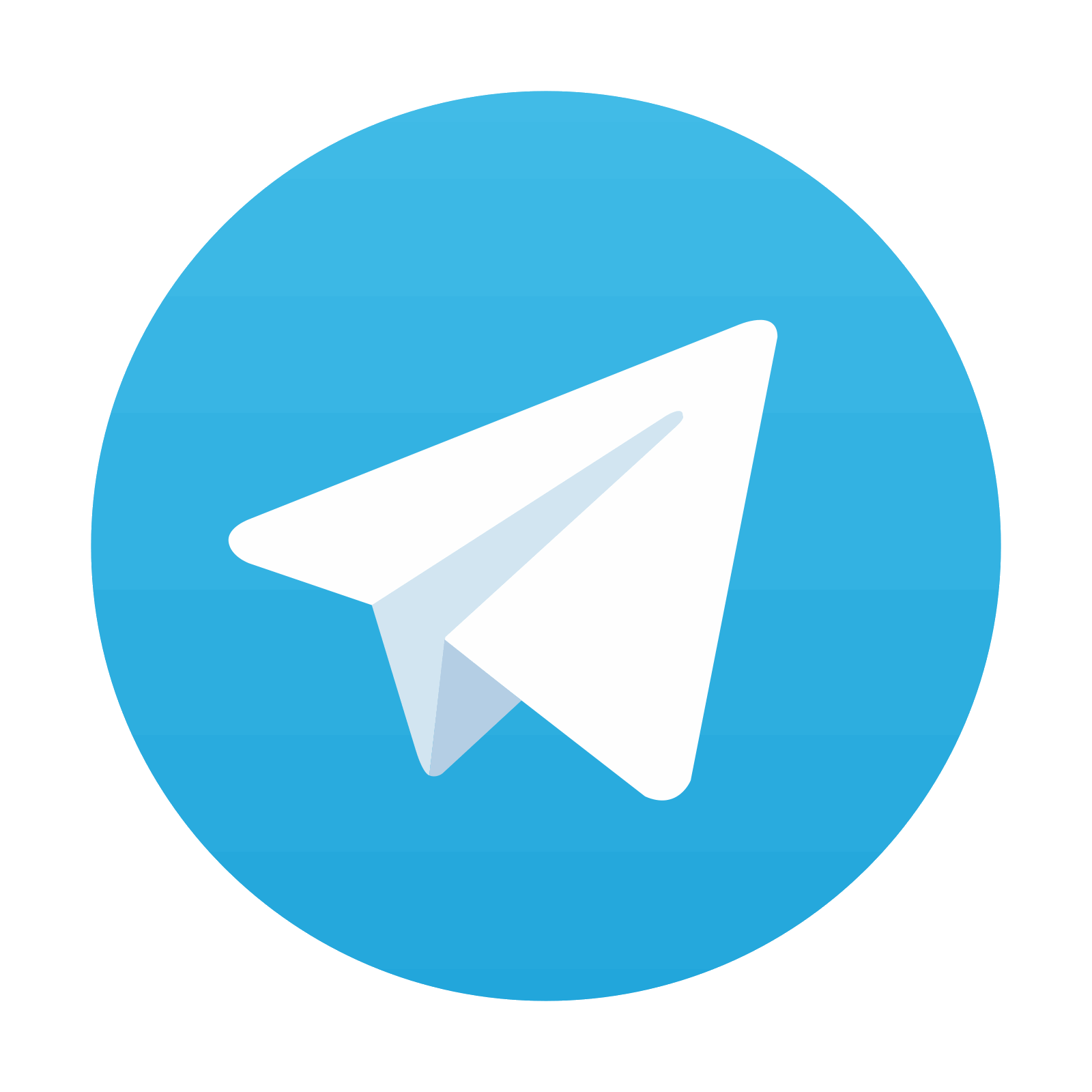
Stay updated, free articles. Join our Telegram channel
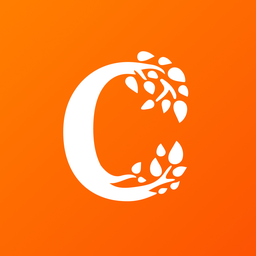
Full access? Get Clinical Tree
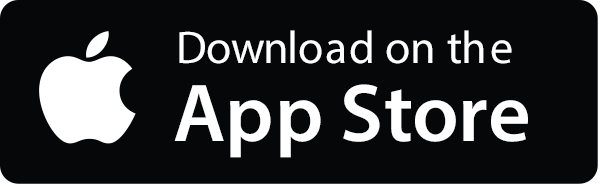
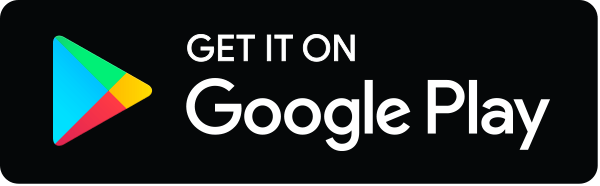