Enterotoxigenic Escherichia coli
James M. Fleckenstein, Washington University School of Medicine, St. Louis, MO, USA
Background
Definition and/or classification
The enterotoxigenic E. coli (ETEC) comprise a diverse pathotype of diarrheagenic organisms that share the ability to produce and effectively deliver heat-labile (LT) and/or heat-stable (ST) enterotoxins to target receptors in the small intestine. While a number of additional virulence traits including both fimbrial and non-fimbrial adhesins have been described, ETEC by definition universally produce one of these toxins.
History
Enterotoxigenic E. coli were discovered in the course of clinical investigation of patients with Vibrio cholerae culture-negative stools presenting with clinical cholera characterized by acute onset of watery diarrhea and severe dehydration (Sack, 2011). In the 1950s De, working in Calcutta, first described patients with clinical cholera whose stool yielded pure cultures of E. coli (then referred to as Bacterium coli) (De et al., 1956). Strains from these patients caused fluid accumulation similar to V. cholerae in the rabbit ileal loop model that De had used previously to identify the cholera toxin (De, 1959), suggesting that these organisms also produced an enterotoxin. Subsequent study by a team of cholera investigators from Johns Hopkins University working at the Calcutta School of Tropical Medicine and the Infectious Disease Hospital in Calcutta in the late 1960s led to definitive identification of enterotoxin-producing E. coli from patients with diarrheal disease clinically indistinguishable from severe cholera (Carpenter et al., 1965; Lindenbaum et al., 1965; Gorbach et al., 1971). Similar to De, these investigators recognized that many patients presenting with cholera-like syndromes had pure cultures of E. coli in their stools, and that these organisms produced a heat-labile filterable enterotoxin (Sack et al., 1971). Analysis of patients presenting with severe acute watery diarrheal syndromes in Bangladesh (Evans and Evans, 1973; Nalin, 1975; Ryder et al., 1976; Sack et al., 1977) later corroborated these early studies.
Soon after the initial discovery of enterotoxin-producing E. coli in patients with severe cholera-like syndromes came reports of travelers’ diarrhea linked to E. coli. While travelers’ diarrhea was clearly a previously known entity, Rowe and Taylor first described an outbreak of diarrhea related to particular E. coli serotype O148:H28 in British soldiers deployed to the United Kingdom of Aden (now Yemen) (Rowe and Taylor, 1969), and interestingly, ETEC of the same serotype was shortly thereafter identified in soldiers deployed to Vietnam (Rowe et al., 1970; DuPont et al., 1971). One of these isolates, B7A, was used in early volunteer challenge studies which firmly established the pathogenic nature of ETEC (Levine et al., 1979). Following these initial reports, came the publication of a number of studies establishing the importance of ETEC as the principal etiologic agent of travelers’ diarrhea (Shore et al., 1974; Merson et al., 1976).
Evolution
Enterotoxigenic E. coli strains exhibit both phenotypic and genetic diversity. This likely relates to the fact that genes for both ST and LT are encoded on plasmids (Gyles et al., 1974). Several lines of evidence support the idea that ETEC have arisen through independent acquisition of these essential toxin genes by a genetically diverse population of E. coli. First, while some serotypes appear more commonly in collections of ETEC than others, enterotoxigenic E. coli are represented by multiple O and H serotypes (Wolf, 1997). Some phylogenetic comparisons based on multi-locus sequence typing (MLST) would likewise suggest that the chromosomal background of ETEC strains is not highly conserved (Turner et al., 2006). Nevertheless, in the most extensive phylogenetic analysis of ETEC to date, performed on over 1000 ETEC isolates, Steinsland et al. determined that strains segregated into distinct clonal groups represented the majority of ETEC (Steinsland et al., 2010). Their analysis suggested that the population of currently circulating ETEC strains likely emerged on several occasions from distinct established globally distributed lineages. One implication of these later studies is that while the evolution of ETEC has certainly been complex, the existence of distinct pathogen lineages may facilitate the identification of conserved antigens useful in vaccine development.
Epidemiology and global impact
Diarrheal illnesses are a leading cause of death in developing countries where more than a fifth of all deaths in children under the age of 5 years can be attributed to infectious diarrhea (Kosek et al., 2003). Collectively, diarrheal pathogens are estimated to cause between 1 and 2 million deaths annually (Kosek et al., 2003; Boschi-Pinto et al., 2008). ETEC contributes significantly to mortality associated with diarrheal illness, accounting for hundreds of thousands of deaths each year. Moreover, these illnesses appear to contribute substantially to overall morbidity and may relate to delayed growth in infected children (Petri et al., 2008).
Traveler’s diarrhea
The diarrheal attack rate among travelers is appreciable with roughly one third to one half (Merson et al., 1976; Sack, 1990) of them becoming ill, often within days of arriving at their destination. Since shortly after ETEC were identified as a causative agent of diarrheal illness, they have been linked to diarrhea in travelers. In virtually every series ETEC is the predominant pathogen accounting for more than half of the cases where an etiologic agent is identified (Black, 1990; Sack, 1990). Largely, this is a reflection of the fact that many travelers under study arrive from regions with adequate sanitation at destinations where sanitation is substandard. Without immunity established by repeated exposure, travelers to developing countries are at high risk for acquisition of ETEC infection as these organisms are ubiquitously distributed in regions where sanitation is poor (Subekti et al., 2003).
Non-governmental organization (NGO) personnel military deployment and diarrhea
Soldiers, NGO personnel, and Peace Corp volunteers deployed to regions where sanitation is often quite poor often fall victim to infectious diarrhea during their deployment. Data from this subset of travelers provide valuable insight into the global epidemiology of infectious diarrheal pathogens as these individuals are often deployed for extended durations to areas not frequented by tourists where local microbiologic data are otherwise lacking (Haberberger et al., 1991; Hyams et al., 1991; Bourgeois et al., 1993). Of the many studies performed in this population, most have also found ETEC to be the predominant pathogen (Riddle et al., 2006).
Seasonality in developing countries
In Bangladesh, there is considerable seasonal variation in ETEC with biannual peaks of illness in the warmer spring and early fall periods (Qadri et al., 2005, 2007). Similarly, diarrheal illness in Egypt has been shown to peak in warmer months of the year (Abu-Elyazeed et al., 1999), and in studies of travelers with diarrhea in Mexico, the rate of ETEC infections increased by 7% for every degree centigrade increase in ambient temperature (Paredes-Paredes et al., 2011).
Emergence of ETEC in developed countries
Interestingly, despite the clear preponderance of ETEC infections in developing regions of the world, a spate of large-scale ETEC outbreaks in the US (Rosenberg et al., 1977; CDC, 1994; Dalton et al., 1999; Beatty et al., 2004; Devasia et al., 2006) attests to the fact that even in industrialized countries these organisms can cause serious harm. While some outbreaks can be traced to importation of food from beyond our borders (MacDonald et al., 1985), in many cases the ultimate source of ETEC is unclear (Beatty et al., 2004). Because screening for ETEC is not routinely performed in clinical microbiology laboratories, these organisms usually escape recognition in sporadic cases and are only identified during the course of investigation of large clusters of patients presenting with diarrheal disease (Jain et al., 2008). On systematic screening of patients with diarrhea, domestically acquired ETEC infections have been identified in studies from Sweden (Svenungsson et al., 2000) and in Minnesota (Beatty et al., 2004), suggesting that these organisms are responsible for some small percentage of sporadic diarrheal illness in industrialized countries including the US. One of the larger recorded outbreaks occurred in the suburban Chicago area when a single delicatessen catered multiple events, disseminating ETEC-laden food, ultimately affecting thousands of people (New Tork Times, 1998; Beatty et al., 2004).
Molecular pathogenesis
Regulation
cAMP receptor protein (CRP) modulation of gene expression in ETEC
Like other pathogens, ETEC respond to their environment by modulating virulence gene expression, often as a result of sensing small molecules such as glucose. Interestingly, some genes such as those encoding LT are optimally expressed in the presence of glucose (catabolite activation), while other genes such as those involved in elaboration of some pili, including CFA/I, are repressed by glucose (catabolite repression). As in other important pathogens (McDonough and Rodriguez, 2012), cyclic AMP appears to play a central role in modulating the expression of these and other ETEC virulence molecules. High levels of glucose inhibit bacterial adenylate cyclase and reduce levels of cAMP and consequently its interaction with the cAMP receptor protein (CRP). In the case of heat-labile toxin, the CRP–cAMP complex represses expression of the eltAB genes encoding LT; eltAB genes are therefore de-repressed or stimulated in the presence of glucose as cAMP intracellular concentrations fall and there is no CRP–cAMP complex to bind to the eltA promoter region and inhibit initiation of transcription by RNA polymerase (Bodero and Munson, 2009) (Figure 6.1).
FIGURE 6.1 Modulation of LT gene transcription by CRP provides an example of catabolite activation in ETEC. (a) At high glucose concentrations, cAMP levels in the cell are low and the homodimeric CRP molecule (shown with four potential cAMP binding sites) is inactive allowing RNA polymerase to interact with the promoter region and initiate transcription of eltA. (b) At low glucose concentrations, cAMP levels in the bacteria increase, activating CRP and permitting the CRP–cAMP complex to bind to a CRP binding site (operator centered at –31.5 upstream from eltA) within the eltA promoter region. This prevents transcription by preventing the RNA polymerase from forming an open complex at the promoter. (Adapted from Bodero and Munson (2009).)
Recent studies in non-pathogenic strains of E. coli demonstrate that more than 100 genes are modulated in response to CRP (Zheng et al., 2004). Similarly, ETEC have adapted this central regulatory mechanism to modulate expression of a number of other virulence genes including tibA (Espert et al., 2011), and the heat-stable toxin gene, estA (Bodero and Munson, 2009) (Table 6.1).
TABLE 6.1
Modulation of virulence determinants by CRP
Gene(s)/ operon | cAMP-CRP modulation | Reference(s) |
eltAB | – | Bodero and Munson, 2009 |
estA | + | Bodero and Munson, 2009 |
fliC | + | Chilcott and Hughes, 2000 |
CS1-3 | + | Evans et al., 1991 |
CFA/I | + | Karjalainen et al., 1991 |
tibDBCA | + | Espert et al., 2011 |
tolC | +∗ | Hantke et al., 2011; Zheng et al., 2004 |
fimA | – | Muller et al., 2009 |
luxS | − | Wang et al., 2005 |
Interestingly, intestinal epithelial cells possess high-affinity cAMP transporters that efflux cAMP into the surrounding milieu (Li et al., 2007) as cAMP levels in the host cell increase. Therefore it is intriguing to hypothesize that ETEC actually sense cAMP generated by target epithelial cells in response to successful delivery of LT, ultimately governing the interaction of ETEC and target epithelium.
Rns and CfaD
Two other transcriptional regulators, Rns (Caron et al., 1989) and CfaD, have been shown to modulate expression of virulence genes in ETEC. Both belong to the AraC/XylS family of transcriptional regulators and their amino acid sequences are 95% identical (Pilonieta et al., 2007). CfaD, located on the large 94.8 kbp virulence plasmid of ETEC strain H10407, has been shown to activate production of CFA/I (Jordi et al., 1992), while Rns is known to activate a number of other genes, including those encoding several other colonization factors (Pilonieta et al., 2007) (Table 6.2).
Not surprisingly, ETEC appear to be programmed to respond to other small molecules that may be found in the small intestine such as those contained in bile. Recent array studies indicate that bile can induce production of both ST and LT (Sahl and Rasko, 2012). Therefore, expression of virulence factors by ETEC likely reflects the integration of multiple signals as these organisms make their way through different environments culminating in epithelial attachment and toxin delivery to the host cell.
Adherence and invasion
Colonization factors
A principal focus of ETEC pathogenesis has been the study of factors which promote colonization of the small intestine. Here, colonization of the intestinal mucosa is thought to be required for efficient delivery of ST and LT (Zafriri et al., 1987; Ofek et al., 1990). Fimbrial structures known as colonization factors (CFs) were among the earliest virulence factors to be identified in ETEC (Evans et al., 1975). Early ETEC volunteer challenge studies demonstrated that a strain cured of a plasmid encoding CFA/I failed to cause diarrheal illness (Figure 6.2a) (Evans et al., 1978; Satterwhite et al., 1978), and recipients of the CFA/I-negative strain had significant reductions in fecal shedding. However, the reduced pathogenicity observed in these studies likely was not simply due to absence of CFA/I as other important determinants are now known to be encoded on this plasmid (Figure 6.2b).
FIGURE 6.2 Importance of a large virulence plasmid in ETEC pathogenesis. (a) Summary of early clinical challenge studies (Evans et al., 1978; Satterwhite et al., 1978) with wild-type ETEC strain H10407, and H10407-P, a spontaneous deletion mutant lacking the large virulence plasmid previously known to encode CFA/I genes. Bar graph shows mean stool volume for H10407 (light blue) and H10407-P (gray); line graph shows recovery of ETEC from stool following challenge. (b) Organization of virulence genes on the large 94.8 kb virulence plasmid of ETEC H10407. In addition to CFA/I genes (yellow) are two putative toxin genes (orange), est1b encoding ST1b and astA encoding EAST1. Also encoded on this plasmid are the EtpBAC two-partner secretion system (dark blue), the eatA autotransporter gene (green), cexE and its presumed secretion system (light blue) and the cfaD regulator gene.
The molecular and structural biology of CFA/I pilus biogenesis has been described in some detail (Jordi et al., 1992; Li et al., 2009). This pilus belongs to the chaperone-usher family of adhesive organelles (see Chapter 12). The plasmid-encoded CFA/I operon encompasses genes for CfaA a putative chaperone, CfaB the major fimbrial structural subunit, CfaC a putative outer membrane usher protein, and CfaE, the minor pilin tip adhesin subunit (Baker et al., 2009). The assembled CFA/I pili are approximately 1 µm in length with approximately 1000 CfaB subunits polymerized into the shaft to present single CfaE subunits as the tip adhesin. Intriguingly, in a spring-like fashion (Mu et al., 2008), CFA/I can assume both tightly wound helical structures or more relaxed open states in response to shear stress, perhaps permitting enhanced adhesion in response to intestinal flow (Andersson et al., 2012).
Plasmid CF loci encode a wide variety of proteinaceous structures that assume the shape of fimbriae, fibrils, helical, or afimbrial surface molecules (Wolf, 1997; Qadri, et al., 2005). Since the discovery of CFA/I, new CFs continue to be identified, with more than 25 antigenically distinct factors described to date (Qadri et al., 2005). On average the fimbrial structures described to date are on the order of 1–2 µm in length. Distinct from these structures is a type IV pilus referred to as longus that extends to more than 20 µm from the bacterial surface (Giron et al., 1994). LngA, the major structural pilin subunit of longus, also known as CS20, is recognized during ETEC infections (Qadri et al., 2000) and shares significant N-terminal homology with other type-4 pilins including those which comprise the toxin co-regulated pilus (TCP) of V. cholerae and the bundle forming pili of enteropathogenic E. coli (see Chapter 13) (Giron et al., 1997).
Interestingly, intestinal colonization and adherence are complex phenotypes that involve multiple genes in addition to the classic colonization factors. Studies implicating LT in promoting epithelial cell adherence (Johnson et al., 2009) and intestinal colonization (Allen et al., 2006), suggest that enterotoxins offer advantages to the bacteria that surpass simple dissemination into the environment.
Type 1 fimbriae
Genes encoding type 1 fimbriae of E. coli are highly conserved (see Chapter 12) and ETEC (Levine et al., 1983; Knutton et al., 1984a, b;), similar to uropathogenic E. coli (UPEC) are known to make these structures. However, relative to the extensive study of the role of type 1 fimbriae in UPEC pathogenesis (see Chapter 9), comparatively little is known about their involvement in ETEC virulence. Despite some early enthusiasm for targeting type 1 pili in combination ETEC vaccines (Levine, 1981), initial efforts to protect against ETEC infections by vaccination with type 1 pili were disappointing (Levine et al., 1982). Nevertheless, tremendous advances in our understanding of the biogenesis of type 1 pili (Waksman and Hultgren, 2009), the very complex nature of their role in UPEC infections (Mulvey et al., 2000; Schilling et al., 2001), and recognized diversity in FimH tip adhesin (Sokurenko et al., 1997) structures could stimulate additional examination of a role for these highly conserved fimbriae in ETEC pathogenesis and vaccine development.
E. coli common pili (ECP)
The genomes of most E. coli encode a variety of potential pilus systems. E. coli common pili (ECP), originally discovered in enterohemorrhagic E. coli (Rendon et al., 2007) and commensal organisms, are also expressed by other E. coli pathotypes, including ETEC (Blackburn et al., 2009). Although at present, the contribution of these structures to the pathogenesis of ETEC is uncertain, they could act in concert with other adhesins as has been noted for EPEC (Saldana et al., 2009).
Outer membrane proteins
Two outer membrane proteins that have been identified in some strains of ETEC promote invasion of intestinal epithelial cell lines in vitro. These include Tia, a 25 kDa (Fleckenstein et al., 1996, 2002) heparin sulfate binding outer membrane protein encoded on a pathogenicity island of ETEC H10407 (Fleckenstein et al., 2000), and TibA (Elsinghorst and Weitz, 1994), a glycosylated autotransporter protein (Lindenthal and Elsinghorst, 1999). Glycosylation of TibA likely requires TibC, a hepatosyltransferase (Moormann et al., 2002). The relevance of epithelial cell invasion per se in the pathogenesis of ETEC remains uncertain, but it is likely that the in vitro invasion phenotype reflects intimate interactions between the bacteria and the host.
Novel antigens
A modified TnphoA mutagenesis strategy to identify secreted or surface-expressed antigens from ETEC led to the identification of a two-partner secretion locus (Fleckenstein et al., 2006) encoded on the large 94.8 kb virulence plasmid of ETEC H10407 that also encodes CFA/I (see Figure 6.2b). Three contiguous genes, etpB, etpA, and etpC encode an outer membrane transport pore (EtpB), a secreted glycoprotein adhesin (EtpA), and a putative transglycosylase (EtpC). Interestingly, EtpA appears to promote ETEC adhesion in a unique fashion by bridging highly conserved flagellin residues exposed only at the tips of ETEC flagella, with receptors on the mucosal surface (Roy et al., 2009).
A similar strategy was also used to identify EatA (Patel et al., 2004), a secreted serine protease autotransporter (SPATE) molecule, which is also encoded on the large virulence plasmid of ETEC H10407, downstream from the etpBAC locus (see Figure 6.2b). Recent studies of EatA have demonstrated that this molecule modulates ETEC adhesion by degrading the EtpA adhesin molecule, in the process promoting effective delivery of the heat-labile toxin (Roy et al., 2011).
Interestingly, searches of potential CfaD binding sites led to the discovery of a novel secreted protein, CexE (Pilonieta et al., 2007), also encoded on the large 94.8 kb virulence plasmid of ETEC H10407, along with a potential secretion locus (see Figure 6.2b). While the function of CexE is uncertain, it is recognized by host antibodies during the course of ETEC infection (Roy et al., 2010).
Damage
The principal effector molecules that define ETEC are the enterotoxins that are secreted by ETEC. These include heat-labile toxin (LT), structurally and functionally similar to cholera toxin, and the small peptide heat-stable toxins (Figure 6.3). Strains may produce any or all of these toxins.
FIGURE 6.3 Toxins produced by ETEC. The ETEC heat-labile toxin (LT) is depicted in (a), with A1 domain shown in blue, and the A2 domain in green, anchoring the A-subunit in the B pentamer. (b) E. coli heat-stable toxins. The predicted structure of the core active toxin domain of ST-P (amino acid residues C5–C17) is shown with sulfur atoms involved in disulfide bond formation in red. Alignments of ST-1a, ST-1b, EAST1 and the native human peptide guanylin are shown below the molecule. LT structure obtained from protein data bank (pdb) entry 1LTB, and rendered in VMD (Humphrey et al., 1996); ST-P was rendered in Protein Workshop (Moreland et al., 2005) from pdb entry 1etn.
Heat-labile toxin (LT)
The heat-labile toxin is a heteroheximeric molecule composed of a B-subunit pentamer and a single A-subunit. Toxin activity resides in the A-subunit, while the B-subunit is largely responsible for host cell binding. The A-subunit is comprised of two principal domains, the A1 domain, responsible for the toxicity, and A2 which non-covalently anchors the A-subunit in the center of the B pentamer (Sixma et al., 1991). Thus the overall structure (Figure 6.3a) has been compared to a ‘ring (the B-pentamer) on a finger’ (the A-subunit) (van Heyningen, 1991).
The B-subunit engages GM-1 gangliosides centered in lipid rafts on the surface of eukaryotic cells to trigger internalization of the toxin into the host cell following proteolytic ‘nicking’ of A1 and A2 into separate polypeptides (of approximately 22 and 5 kDa, respectively) by serine proteases located at the apical surface of epithelial cells (Lencer et al., 1997). The subunits remain joined by a single disulfide bond following cleavage (Lencer et al., 1997). Following uptake of the A1 subunit into the host cell cytoplasm, it acts in concert with cellular ADP ribosylating factors (ARFs) to transfer ADP-ribose from NAD to the alpha subunit of the heterotrimeric GTPase, (Gsα) (Spangler, 1992). Formation of a ternary complex of ADP-ribosylated Gsα GTP and adenylate cyclase catalyses cAMP production from ATP. The resulting increases in intracellular concentrations of cAMP activate protein kinase A, which subsequently phosphorylates the cystic fibrosis transmembrane conductance regulator (CFTR) activating the chloride channel (Cheng et al., 1991). This promotes efflux of chloride into the intestinal lumen paralleled by net decreases in Na+ and water absorption resulting in watery diarrhea characteristic of ETEC.
LT secretion requires a functional type II secretion system
Secretion of LT from ETEC requires the presence of a functional type II secretion system (see Chapter 13), similar to the secretion apparatus for CT in Vibrio cholerae (Tauschek et al., 2002). In the protypical ETEC strain H10407, LT secretion appears to be further modulated by one or more genes encoded by a pathogenicity island including leoA (Fleckenstein et al., 2000), a GTPase (Brown and Hardwidge, 2007). Interestingly, much of the LT secreted by ETEC in vitro remains associated with lipopolysaccharide of outer membrane vesicles produced by the bacteria (Horstman and Kuehn, 2000).
Heat-stable toxins
ETEC may secrete one or more plasmid-encoded heat-stable toxins (ST), small cysteine-rich peptides that engage an extracellular domain of guanylyl cyclase C (GC-C) on the apical surface of intestinal epithelial cells (Potter, 2011). This binding triggers activation of the intracellular portion of GC-C which converts GTP to the intracellular messenger cGMP. Elevations in cGMP lead to activation of the membrane-bound (French et al., 1995; Vaandrager et al., 1998) cGMP-dependent protein kinase (cGK II or PKG) that in turn phosphorylates CFTR (Pfeifer et al., 1996; Vaandrager et al., 2000).
Several different variants of ST have been described in human strains. STa (STI) peptides come in two varieties ST-Ia(ST-P) and ST-Ib(ST-H) (Figure 6.3b), both of which are plasmid-encoded, typically on transposition elements. These peptides, as well as a similar native peptides, uroguanylin and guanylin, both activate GC-C, also known as the STa receptor or STaR (Chao et al., 1994; Giannella and Mann, 2003). Both ST1 molecules are produced as pro-peptides that undergo processing and export into the periplasm. Export of ST1b, and presumably ST1a, through the outer membrane of E. coli depends on the TolC (Yamanaka et al., 1998) type 1 secretion system (see Chapter 16). In contrast, STb peptides have also been identified in human strains of ETEC, but predominately are found in porcine strains, and do not bind GC-C or activate cGMP, and unlike the STa (Sack et al., 1975; Levine et al., 1977) peptides STb toxins are not as clearly linked to human illness (Weikel et al., 1986).
EAST1, or EnteroAggregative heat-Stable Toxin (Savarino et al., 1993), has predicted structural similarity to STI molecules (Figure 6.3b), and is encoded by ast genes residing on mobile elements (McVeigh et al., 2000) of plasmids in some ETEC strains (Savarino et al., 1996; Yamamoto and Echeverria, 1996). While the functional significance of these genes has yet to be determined, they do appear to encode a molecule with the capacity to stimulate cGMP production in vitro. Some strains, such as the prototypical ETEC strain H10407, encode LT, ST1a, ST1b, and EAST1 (Fleckenstein et al., 2010) suggesting substantial functional redundancy in the production of enterotoxins.
Candidate virulence molecules of unknown function
Subtractive DNA hybridization studies (Chen et al., 2006), the completion of multiple ETEC genomes (Rasko et al., 2008; Crossman et al., 2010; Sahl et al., 2011), and proteomic studies (Roy et al., 2010) have identified a variety of other molecules that may be important in the pathogenesis of ETEC and provide candidate targets for vaccine development. Studies currently underway are attempting to examine the utility of some of these molecules (Harris et al., 2011), and to examine their function.
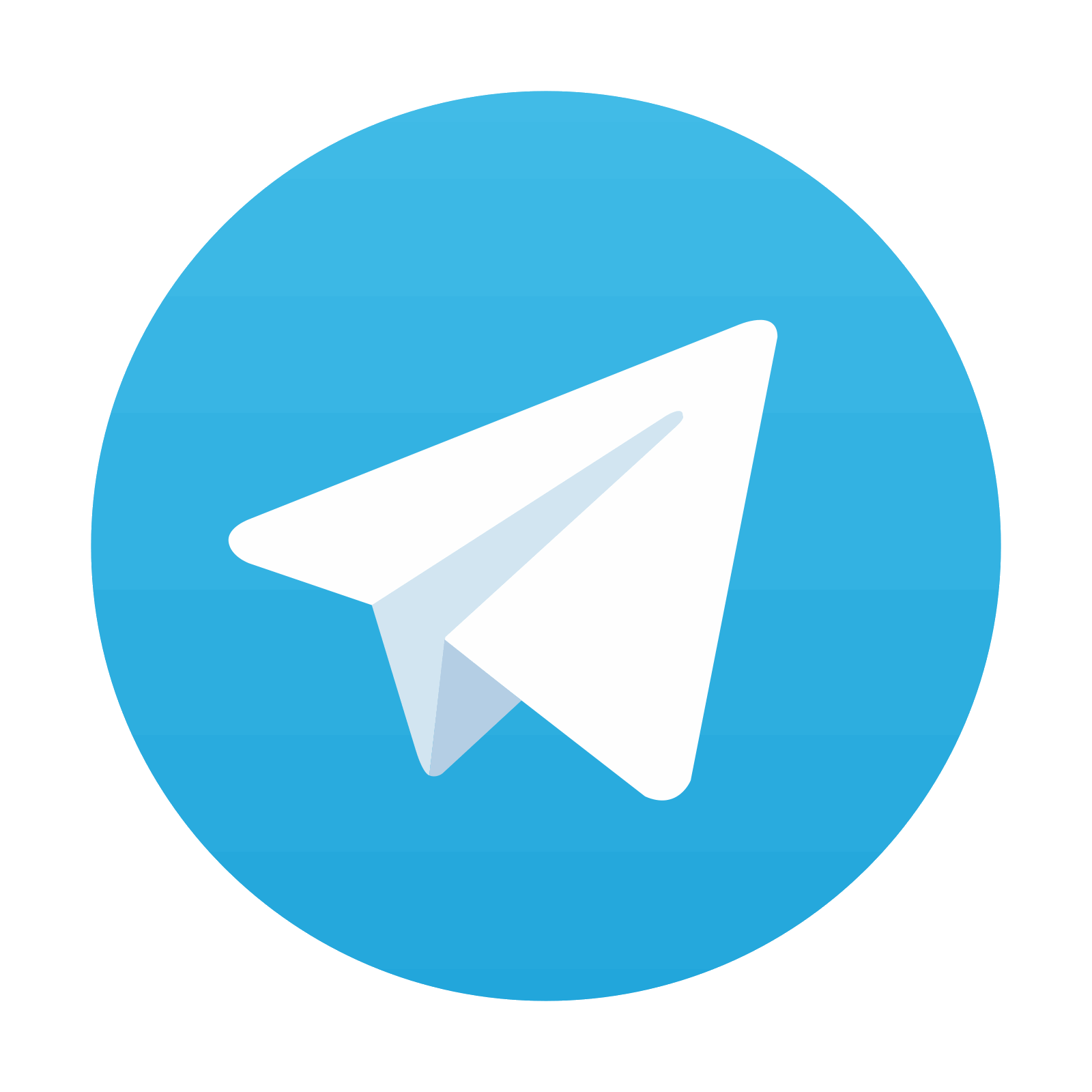
Stay updated, free articles. Join our Telegram channel
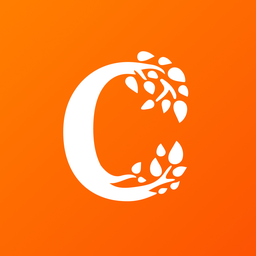
Full access? Get Clinical Tree
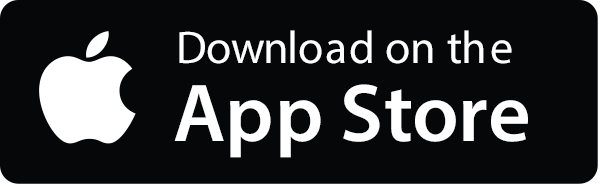
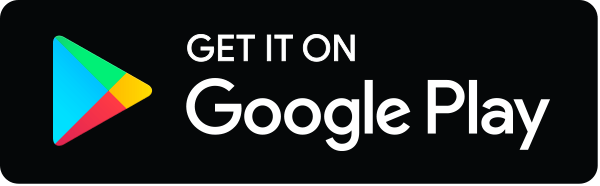