Fig. 22.1
a TEM image of ~8 nm-sized β-NaLuF4:Gd/Yb/Tm. b In vivo imaging of a black mouse after subcutaneous injection of citric acid-modified sub-10 nm-sized β-NaLuF4:Gd3+/Yb3+/Tm3+ and citric acid-modified β-NaYF4:Yb/Tm when detected from the chest side (left) or the back side (right). c Left TEM image of β-NaYF4:Yb/Er. Right The time trace of emission intensity from a single UCNP under continuous laser illumination for over 1 h (Recreated with kind permission of American Chemical Society [29, 46])
Besides improving the optical property of small UCNP either by altering the doping strategy or changing the crystal hosts, epitaxial growth of a protective shell represents the second technique for reducing the surface defects and improving the UCL property [30, 31]. However, successful synthesis of sub-10 nm-sized core@shell-structured UCNP has not been achieved until very recently. By changing the concentration of basic surfactants, Y3+:F− ratio and reaction temperature, Ostrowski et al. have succeeded in synthesizing core@shell-structured hexagonal-phase NaYF4:Yb/Er@NaYF4 with a diameter less than 10 nm [32]. As-synthesized smaller UCNP were extremely stable and exhibited no measurable photobleaching or blinking (Fig. 22.1c).
Although, the engineering of ultrasmall UCNPs for biological imaging and therapy is still at its early stage, they are expected to play a vital role in designing tumor-targeted imaging probe that could be efficiently cleared from body. To date, most of researchers are still interested in developing core@shell-structured UCNPs with particle size ranging from 10 to 50 nm for biological imaging applications.
22.3 Engineering of UCNP for Biological Imaging
22.3.1 Engineering the Surface for Water-Soluble UCNPs
With the superior UCL properties and suitable surface modification and functionalization, UCNP has been demonstrated as a promising candidate of traditional imaging agents (e.g., QDs and organic dyes). Surface engineering is the first and a vital step before any biological imaging of UCNP. Although oleic acid-assisted strategy could guarantee high-quality UCNPs with uniform size and morphology, as-synthesized UCNPs are always coated with a layer of oleic acid ligands, which makes UCNPs water insoluble. So far methods used for engineering water-soluble UCNPs include ligand exchange (or removal) [33, 34], ligand oxidation reaction [35], layer-by-layer method [36], hydrophobic–hydrophobic interaction [37], host–guest interaction [38], and silanization [39].
22.3.2 Homemade In Vivo Imaging System for UCNP
One of the obstacles in developing UCNP for biological imaging applications lies in the lack of 980 nm excitation source in nearly all of the commercially available confocal microscopes and in vivo imaging systems. To date, all of the in vitro and in vivo UCNP imaging researches are completed by using homemade (or modified) imaging systems [5, 6, 40].
Nyk et al. reported the first whole-body in vivo imaging of UCNP in mouse [40]. As-synthesized UCNPs (i.e., NaYF4:Tm/Yb) were excited at 975 nm by a defocused emission from a fiber-coupled laser diode. An emission filter (cut off at 850 nm) was used to keep the 975 nm excitation light of the imaging CCD camera. Images were collected and processed by using Maestro GNIR FLEX fluorescence imaging system (CRi). After dosing the Balb-c mouse with NaYF4:Tm/Yb (200 μL of 2 mg/mL in water with 5 % glucose), a high-contrast signal from UCNPs could successfully be detected both through the skin and after dissection of the animal (Fig. 22.2a).
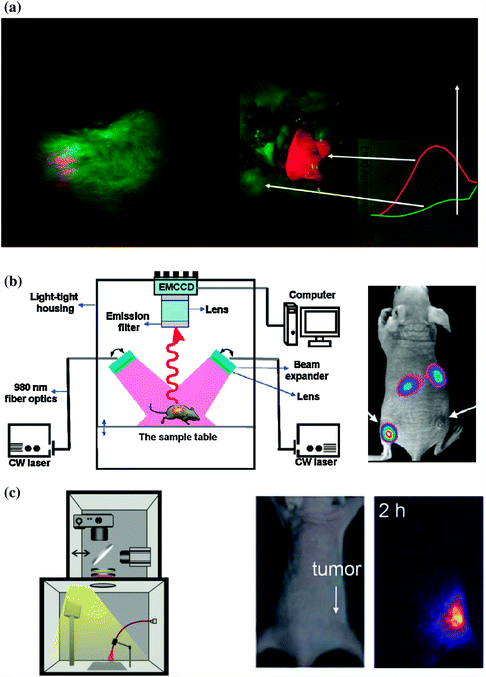
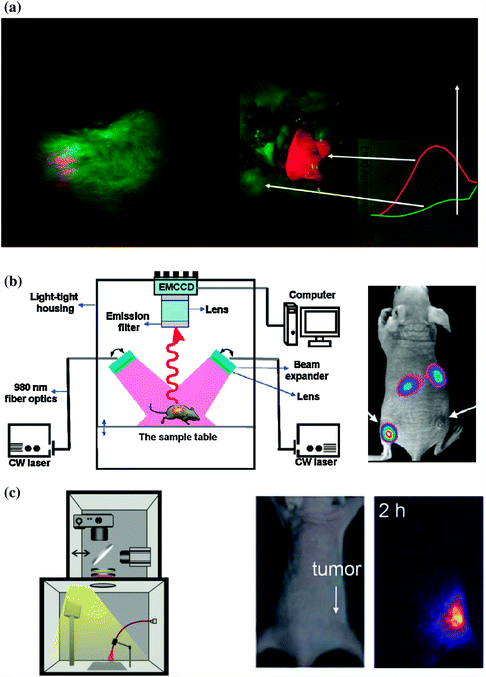
Fig. 22.2
Comparison of different homemade UCNP in vivo imaging systems. a In vivo whole body images of mouse injected with UCNPs. Images were obtained in a modified Maestro GNIR FLEX fluorescence imaging system (CRi). (left) Intact mouse, (right) same mouse after dissection. b Left Schematic illustration of an in vivo UCNP imaging system based on modification of a Kodak in vivo imaging system. Right A representative images showing the in vivo tumor-targeted imaging of U87MG and MCF-7 tumors bearing mouse. c Left In vivo luminescence imaging setup which composed of a fiber-coupled 980 nm laser, a DSLR camera, optical filters, and a dark box. Middle: bright filed image of mouse. Right UCL image of the tumor site from mouse (Recreated with kind permission of American Chemical Society [10, 40] and Wiley–VCH Verlag GmbH & Co. KGaA [41])
In a separated study, Xiong et al. reported an improved UCNP in vivo imaging setup by adding two CW 980 nm lasers, Andor DU897 EMCCD and filters to a Kodak in vivo imaging system [10]. The successful in vivo UCNP imaging has been demonstrated in U87MG and MCF-7 tumors bearing nude mice. After intravenous (i.v.) injection of RGD-labeled NaYF4:Er/Tm/Yb (~50 μg/mouse), the mice were illuminated with two beam-expanded 980 nm lasers. A cooled EMCCD camera was then used to record the 800 nm emission of UCNPs and images were processed using Kodak molecular imaging software. As shown in Fig. 22.2b, under excitation of 980 nm laser, intense UCL signal could be observed in the U87MG tumor, while no significant signal could be seen in the MCF-7 tumor, indicating the targeted tumor uptake of UCNPs in U87MG. A high signal-to-noise (~24) between tumor and the background has been achieved in this study.
Besides modifying commercially available in vivo imaging systems by adding extra 980 nm laser and suitable filters, combining digital single-lens reflex (DSLR) camera and suitable optical filters might represent the simplest way to carry out an in vivo UCNP imaging experiment [41, 42]. Park et al. have built an in vivo UCNP imaging system by using a fiber-coupled 980 nm laser, a Nikon D90 DSLR camera, certain optical filters, and a dark box. The authors managed to image the tumor uptake of UCNP-Ce6 in nude mouse with their system [41]. Possibly because of the limited light coverage of 980 nm laser on mice, only signal from tumor site could be seen from the images (Fig. 22.2c). Also, since green light from UCNP could only have very limited tissue penetration depth, better in vivo images could be achieved by altering the system for detecting strong NIR (800 nm) or red (657 nm) emissions from UCNP.
22.3.3 Engineering of UCNP for Excellent NIR and Red Emissions
22.3.3.1 Strategies for Improving the NIR-Band Emission
Red and NIR light could have much deeper tissue penetration depth compared with UV and other visible light (e.g., blue and green light). Typically, UCL of UCNP is known to have multiple emission bands with various optical intensities after 980 nm excitation. For example, the emissions of NaYF4:Er/Yb/Tm nanoparticles include UV (330–350 nm, 350–370 nm), blue (390–420 nm, 440–460 nm, 460–500 nm), green (510–530 nm, 530–570 nm), red (630–660 nm), and NIR (770–810 nm) bands [17]. Recently, there has been an increasing focus on the design and synthesis of UCNPs with tunable UC emission from UV to near-infrared (NIR) through various doping strategies or by introducing well-designed core@shell nanostructures [40, 43]. In particular, engineering of UCNPs with excellent NIR emission (λ = ~800 nm) or single-band red emission (λ = ~650 nm) could result in ideal optical biolabels for future deep tissue imaging and has become a new attractive topic currently [44].
The mostly used UCNP for achieving a NIR-to-NIR in vivo imaging is Tm3+ and Yb3+ co-doped β-NaYF4, which, after 980 nm excitation, could give out strong NIR emission located at 800 nm, ideally for deep tissue imaging [8, 40]. Several interesting methods have been developed to improve the NIR emission intensity of NaYF4:Tm/Yb nanoparticles.
Increasing the concentration of Tm3+ ions in the host lattice is one of the simplest techniques to achieve the goal. Wang et al. demonstrated that NaYF4:Tm/Yb nanoparticles with Tm3+ doping fixed at 0.2 mol % could only have a weak emission at 800 nm, and increasing the Tm3+ concentration up to 2 mol % could result in a significant enhancement thanks to the enhanced 3H4 level [45]. By further replacing all Y3+ in NaYF4:Tm/Yb to Yb3+ ions, Chen et al. have developed ultrasmall-sized (7–10 nm) NaYbF4:Tm (2 mol %) nanoparticles with 3.6-fold enhancement in total upconversion emission intensity in comparison with that of 25 nm-sized NaYF4:Tm/Yb (2/20 mol %) [46]. Such enhancement has been suggested to arise from the increased absorptions induced by the Yb3+ ions and the increased efficiency of energy transfer between Yb3+ and Tm3+ ions. In a followed-up study, Liu et al. reported a lutetium (Lu)-based UCNP with excellent NIR emission [29]. As-synthesized sub-10 nm-sized β-NaLuF4:Gd/Yb/Tm (24/20/1 mol %) displayed bright UCL with high QY estimated to be 0.47 ± 0.06 %. In comparison with 20 nm-sized NaYF4:Tm/Yb (1/20 mol %), approximately 11-fold enhancement in NIR emission could be achieved. The successful suppression of nonradiative process has been suggested as the main reason behind such enhancement.
Epitaxial growth of an inert protective shell over pre-prepared NaYF4:Tm/Yb core has been accepted as another useful strategy for minimizing the surface defects for preserving the optical integrity of UCNPs [30, 31]. Although cation-exchange and Ostwald ripening methods have been developed as alternative options recently [47, 48], seed-mediated growth still remains the most adopted technique for synthesis of such core@multi-shell structured UCNP [23]. By coating α-NaYbF4:Tm (0.5 mol %) with a well-selected CaF2 protective shell, Chen et al. have developed a novel and biocompatible α-NaYbF4:Tm (0.5 mol %)/Yb@CaF2 with highly efficient NIR-to-NIR upconversion property (Fig. 22.3a) [49]. CaF2 was chosen as the epitaxial shell due to its low lattice mismatch with α-NaYbF4:Tm, good optical transparency, high crystallizability, stability, and biocompatibility. As-design UCNP exhibited an impressive high QY of 0.6 ± 0.1 % under low power density excitation (0.3 W/cm2). Thanks to the suppressing of surface quenching effects via hetero-epitaxial growth of CaF2 shell, an approximately 35-fold enhancement in UCL intensity could be achieved in comparison to the noncoated α-NaYbF4:Tm (Fig. 22.3b). The authors also demonstrated the possibility of imaging deep tissue by using α-NaYbF4:Tm(0.5 mol %)/Yb@CaF2 nanoparticles. Clear NIR signal could be detected even through 3.2 cm pork tissue (Fig. 22.3c).
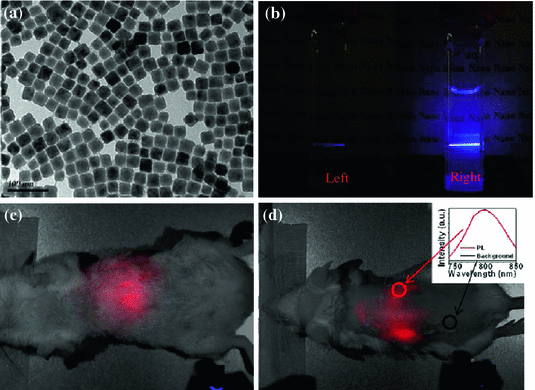
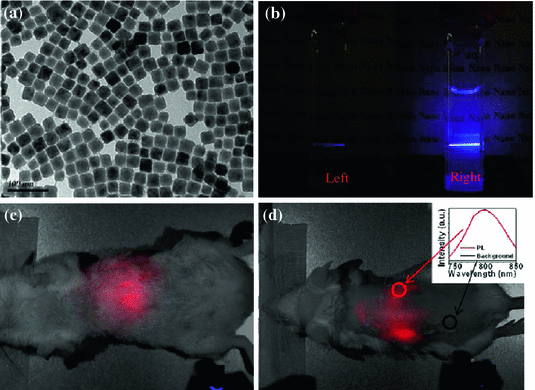
Fig. 22.3
a TEM image of ~27 nm-sized α-NaYbF4:Tm(0.5 mol %)/Yb@CaF2. b Photographic images of cuvettes with suspensions of the core (left) and the core/shell (right) nanoparticles under laser excitation at 975 nm. Whole-animal imaging of a BALB/c mouse injected via tail vein with the HA-coated α-NaYbF4:Tm(0.5 mol %)/Yb@CaF2 core/shell nanoparticles. Mice were imaged in the c belly and d back positions. Insert in d is the spectra of the NIR upconversion luminescence and background taken from the circled area (Recreated with kind permission of American Chemical Society [49])
22.3.3.2 Strategies for Improving the Red-Band Emission
UCNP with strong single-band red emission has been emerged as another interesting imaging probe for in vivo imaging. Recently, studies on Yb3+/Er3+ co-doped MnF2 and KMnF3 nanoparticles have demonstrated a substantially enhanced red-to-green (R/G) emission ratio owing to the energy transfer between the Er3+ and Mn2+ [50, 51]. Inspired by these, Wang et al. have developed cube-shaped KMnF3:Yb/Er (18/2 mol %) with extremely high red emission and completely disappeared blue and green emissions (Fig. 22.4a, b) [44]. Such single-band upconversion emission has been ascribed to nonradiative energy transfer from the 1H9/2 and 4S3/2 levels of Er3+ to the 4T1 level of Mn2+, followed by back-energy transfer to the 4F9/2 lever of Er3+. Pure NIR emission of KMnF3:Yb/Tm (18/2 mol %) could also be achieved by applying the same idea (Fig. 22.4c). In a followed-up study, Tian et al. presented a hydrothermal synthesis of Mn2+-doped NaYF4:Yb/Er nanoparticles with a strong single-band red emission [42]. Surprisingly, as-designed nanoparticles could have a R/G ratio of 163.78 after increasing the doping level of Mn2+ up to 30 mol %. The doping of Mn2+ also resulted in significant enhancement (about 15 times) in overall optical intensity in comparison with Mn-free sample (Fig. 22.4d). As a proof-of-concept experiment, the author injected water-soluble PEGylated NaYF4:Yb/Er/Mn at the foot, back, and upper leg regions of white Kunming mice and demonstrated a clear tissue penetration and high-contrast photoluminescent imaging of the red emission (Fig. 22.4e).
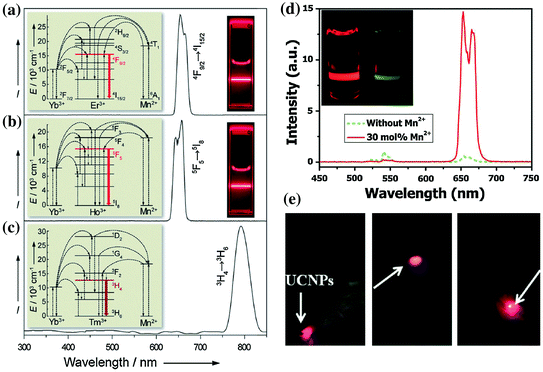
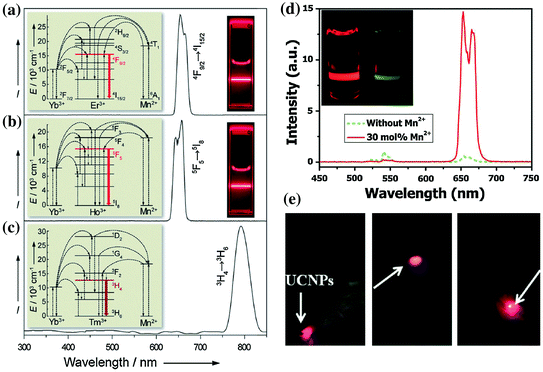
Fig. 22.4
Room-temperature upconversion emission spectra of a KMnF3:Yb/Er (18:2 mol %), b KMnF3:Yb/Ho (18:2 mol %), and c KMnF3:Yb/Tm (18:2 mol %) nanocrystals in cyclohexane (insets proposed energy transfer mechanisms and corresponding luminescent photos of the colloidal solutions). d Upconversion emission spectra of NaYF4:Yb/Er (18/2 mol %) nanocrystals with 0 and 30 mol % Mn2+ dopant ions dispersed in cyclohexane (1 mg/mL), respectively. (inset the corresponding luminescent photographs for intensity comparison). e In vivo upconversion luminescence imaging of PEG-UCNPs injected into translucent foot (left), skin of back (middle), and thin muscles of mice (right) (Recreated with kind permission of Wiley–VCH Verlag GmbH & Co. KGaA [42, 44])
The engineering of UCNP nanostructures for excellent NIR- and red-band emissions by doping with suitable amount of Mn2+ ions will continue to attract increasing interests. More importantly, selectively doping UCNP with other lanthanide ions, such as Gd3+, Lu3+, Yb3+, and 153Sm3+ might endow UCNP with useful imaging modalities, such as magnetic resonance imaging (MRI), CT, and positron emission tomography (PET).
22.3.4 Engineering of UCNP for Multimodal Imaging
Each imaging modality has its strengths and weaknesses [52–55]. MRI provides excellent spatial resolution, exceptional anatomical information, and unlimited depth for in vivo imaging, but suffers from limited sensitivity. PET possesses remarkable detection sensitivity but only with a very limited spatial resolution (~mm). Photoluminescence imaging is capable of providing the highest spatial resolution and is the best modality for live cell imaging. However, it lacks the capability to obtain anatomical and physiological detail in vivo owing to limited penetration of light in tissues. Engineering of UCNP by suitable doping strategy could have a great possibility to achieve a multimodal imaging probe which holds balance in sensitivity, resolution, and penetration depth, enabling excellent visualizing (cancer) cells from the cellular scale to whole-body noninvasive imaging. In this section, we review the recent progress on how to engineer UCNP for in vivo multimodal imaging by lanthanide ions doping strategy.
22.3.4.1 Engineering of UCNP for UCL/MR Dual-modal Imaging
Although combination of super paramagnatic iron oxide nanoparticles (SPIONs) with UCNP could result in bimodal imaging probe with UCL and MR modalities [39, 56], doping paramagnetic Gd3+ ions (or Mn2+) in UCNP crystal lattice should represent a better strategy for introducing MR contrast imaging capability without sacrificing the UCL intensities [11, 12]. Kumar et al. reported the first such example by doping Gd3+ ions into NaYF4 and demonstrated the combination of UCL and MR bimodal imaging in one single nanocrystal [12]. The first in vivo demonstration of such bimodal UCL/MRI imaging was later achieved using hexagonal-phase carboxylic acid-functionalized NaGdF4:Tm3+/Er3+/Yb3+ nanoparticles [13]. However, because the majority of the Gd3+ ions are doped into the rigid crystal lattice and have limited chance to alter the relaxation time of surrounding water protons, only low MR sensitivity could be achieved in most of the previous reports [7, 12, 57]. The nanostructure re-engineering and relaxivity mechanism probing of the Gd3+-doped UCNP might hold a great chance to enhance its MR sensitivity.
Recently, we have designed three different types of Gd3+-doped UCNPs with various Gd3+ ion locations and surface densities to probe the roles of surface and bulk Gd3+ ions on shortening the T 1-relaxation time of surrounding water molecules [11]. Our systematic research have demonstrated that it is only the surface Gd3+ ions in Gd3+-doped UCNP are responsible for shortening the T 1-relaxation time of water protons, and bulk Gd3+ ions could have little impact even with high concentration (Fig. 22.5). A remarkable increase in r 1-relaxivity (up to 6.18 mM−1s−1/Gd3+) has been achieved by further decreasing the NaGdF4 shell thickness down to <1 nm [11]. By using ultrasmall NaGdF4 nanocrystals as models, Johnson et al. have shown the similar observation of increased r 1-relaxivity per Gd3+ ion (from 3.0 to 7.2 mM−1s−1) with decreased NaGdF4 particle size (from 8 to 2.5 nm), again demonstrating the role of surface Gd3+ ions in relaxivity enhancement [58].
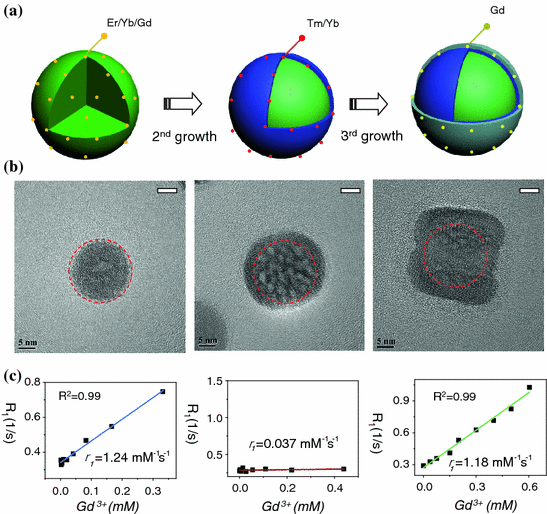
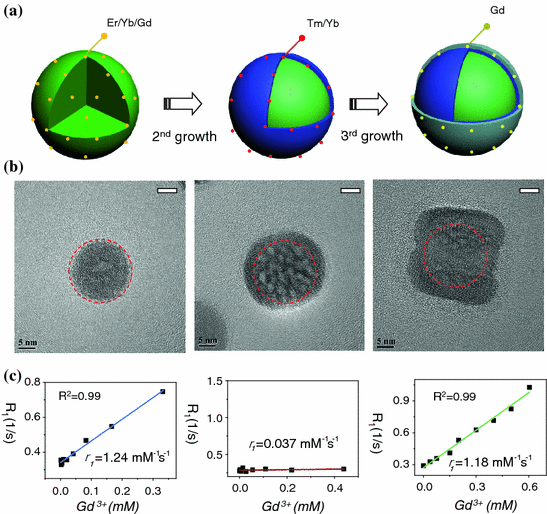
Fig. 22.5
a A schematic illustration showing the synthesis of core@multi-shell UCNPs. b HR-TEM images of highly crystalline NaYF4:Er/Yb/Gd (left), NaYF4:Er/Yb/Gd@NaYF4:Tm/Yb (middle), NaYF4:Er/Yb/Gd@NaYF4:Tm/Yb@NaGdF4 (right) nanoparticles. c Plots of longitudinal relaxation rate (R 1) versus Gd3+ concentration of three samples, (left) NaYF4:Er/Yb/Gd@SiO2 (r 1 = 1.24 mM−1s−1), (middle) NaYF4:Er/Yb/Gd@NaYF4:Tm/Yb@SiO2 (r 1 = 0.037 mM−1s−1), and (right) NaYF4:Er/Yb/Gd@NaYF4:Tm/Yb@NaGdF4@SiO2 (r 1 = 1.18 mM−1s−1) (Recreated with kind permission of Wiley–VCH Verlag GmbH & Co. KGaA [11])
In an attempt to understand the relaxivity mechanism of Gd3+-doped UCNP, we further introduced mesoporous and dense silica coatings for systematically investigating its possible relaxivity mechanisms [59]. A surface-dependent relaxivity mechanism has been proposed based on our results. Specifically, we showed a co-existing of inner- and outer-sphere r 1-relaxivity mechanism in ligand-free Gd3+-doped UCNP, while only main contribution of outer-sphere mechanism in silica-shielded probes. The origin of r 2-relaxivity is inferred mainly from an out-sphere mechanism with the r 2 value being surface state related. Also, key factors for tuning r 2/r 1 ratio have been found to be highly dependent on the thickness of NaGdF4 shell and surface modification strategy. Based on these, we showed a 105-fold enhancement in r 1-relaxivity (14.73 mM−1s−1/Gd3+) in comparison with the first-reported same value (0.14 mM−1s−1/Gd3+). These new findings provide not only deeper insight into the origin of contrast enhancement of water-soluble Gd3+-doped UCNP, but also useful strategy for achieving both high MR sensitivity for future biological imaging applications.
22.3.4.2 Engineering of UCNP for UCL/MR/CT Triple-modal Imaging
With the presence of heavy metal ions like Gd3+, Yb3+, and Lu3+ in NaYF4 matrix, lanthanide ions-doped UCNPs also have been considered as an inherent CT contrast agent recently [14–16, 60]. Ultrasmall NaGdF4:Er/Yb nanocrystals have firstly been developed via an interesting two-phase system and been demonstrated as UCL/MR/CT triple-modal imaging contrast agent [14]. In a followed-up study, Liu et al. reported a Yb-based NaYbF4:Er/Gd nanoparticles with improved CT contrast capabilities [15]. The doping of Gd3+ ions has been demonstrated vital to control both the morphology and R/G ratio of NaYbF4:Er/Gd nanoparticles (Fig. 22.6a, b). As-synthesized water-soluble polyethylene glycol (PEG)-modified NaYbF4:Er/Gd nanoparticles have been found to be the best CT contrast agents among iobitridol, Au-, Pt-, Bi-, and Ta-based nanoparticulate agents under the same testing conditions. In vivo imaging study demonstrated the capability of PEG-modified NaYbF4:Er/Gd nanoparticles as an excellent CT contrast agent (Fig. 22.6c, d). Water-soluble NaLuF4:Tm/Yb nanoparticles might represent the third type of inherent CT agents. After coating with iron oxide shell, or modified with Gd3+-complex, such modified NaLuF4:Tm/Yb nanoparticles could also be probes with well-combined UCL/MR/CT triple modalities [16, 61, 62]. Although other matrixes, such as BaYbF5 and BaGdF5 [63, 64], have also been reported as inherent CT contrast agents, they might not be a good choice for efficient upconversion optical imaging.
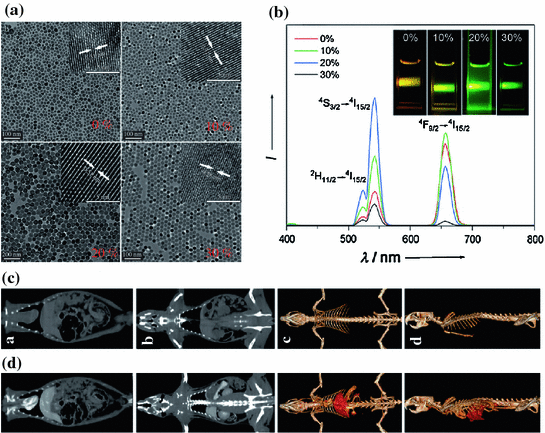
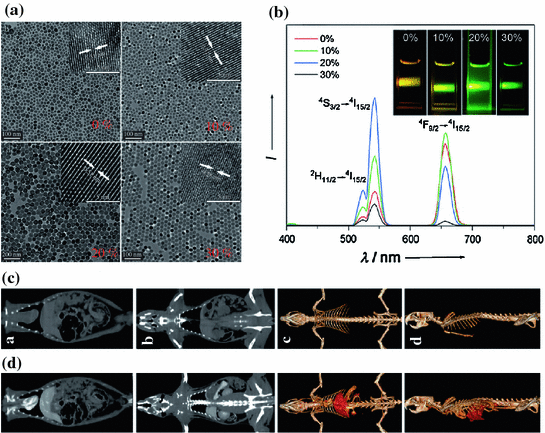
Fig. 22.6
a TEM image of Gd-doped NaYbF4:Er nanoparticles with various doping levels of Gd3+ ions and corresponding b room temperature NIR-to-Vis upconversion luminescence spectra. The inset in b shows the upconversion luminescence photograph of the Gd-doped NaYbF4:Er nanoparticles with different concentrations of Gd under excitation at 980 nm. In vivo CT coronal view images of a rat after intravenous injection of 1 mL PEG-UCNPs (70 mgYb/mL) solution at different time intervals. c Pre-injection, d 10 min postinjection (Recreated with kind permission of Wiley–VCH Verlag GmbH & Co. KGaA [15])
Another way for adding a CT contrast capability is by decorating Gd3+ ions-doped UCNP with CT contrast nanoparticles, such as tantalum oxide (TaOx) and ultrasmall gold nanoparticles (Fig. 22.7) [60, 65]. Starting from NaYF4:Yb/Er/Tm@NaGdF4 nanocrystals, we recently demonstrated an efficiently way to add CT imaging modality by decorating with a fluorescence-transparent TaOx shell [65]. As-design water-soluble litchi-shaped trimodal imaging probe showed an extraordinary high r 1 (11.45 mM−1s−1) and r 2 (147.3 mM−1s−1) values thanks to the ultrathin NaGdF4 shell. An obvious CT contrast enhancement could be obtained by the combined effect of radiopaque TaOx shell and UCNP itself. Both the in vitro and in vivo trimodal imaging have been demonstrated, providing us a new possibility for potential early cancer detection.
22.3.4.3 Engineering of Radiolabeled UCNP for PET/SPECT Imaging
Suitable surface engineering and isotopes labeling of UCNP could make it also a PET/SPECT (i.e. single-photon emission computed tomography) tracer. To date, research on synthesis and application of radioactive UCNP (r-UCNP) is still in its infancy. Two techniques have been developed for the synthesis of radioactive UCNP. They are surface ion reaction (or exchange) method and direct isotope(s) doping strategy.
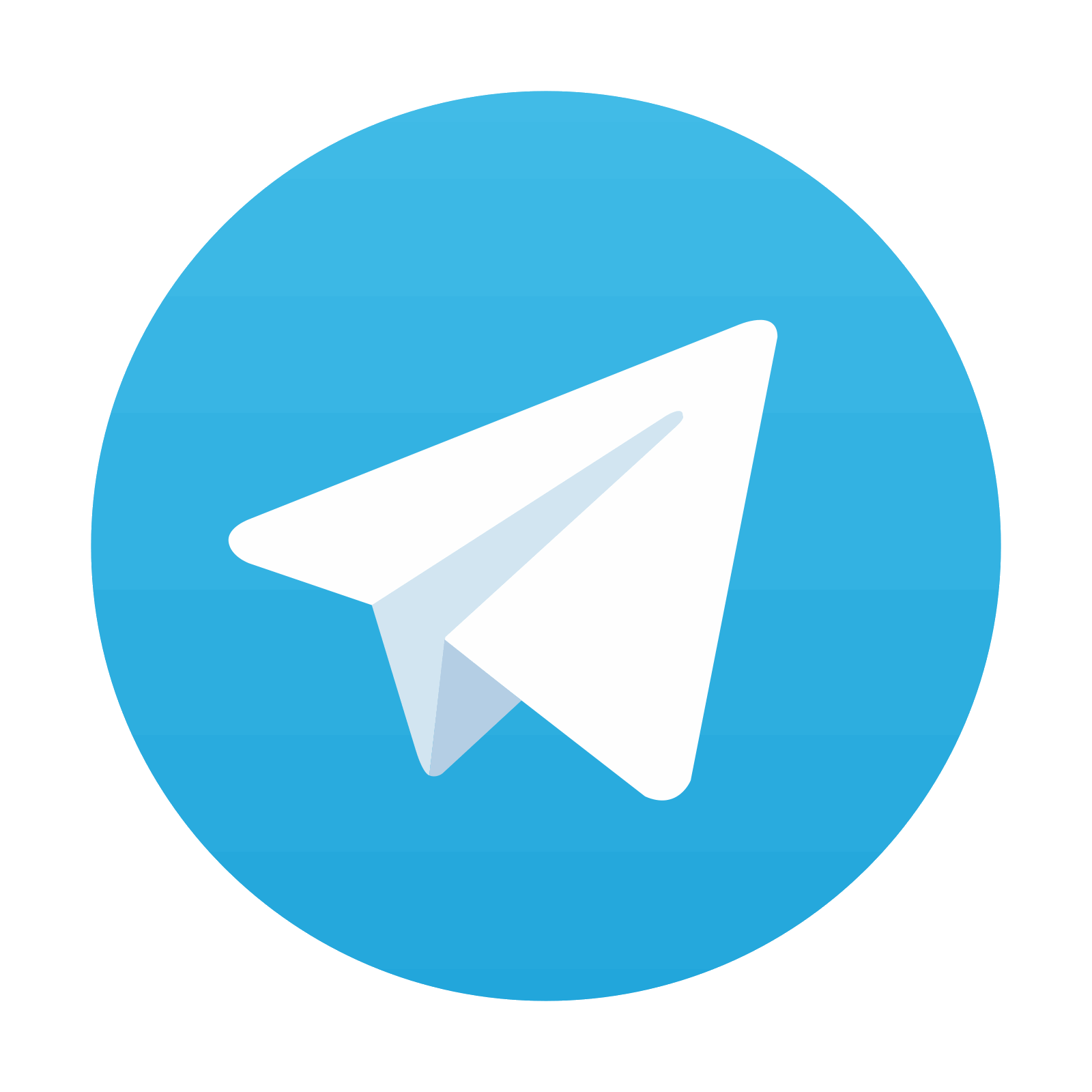
Stay updated, free articles. Join our Telegram channel
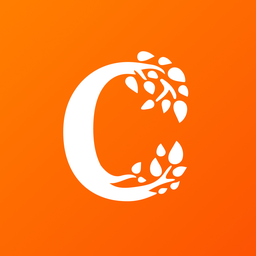
Full access? Get Clinical Tree
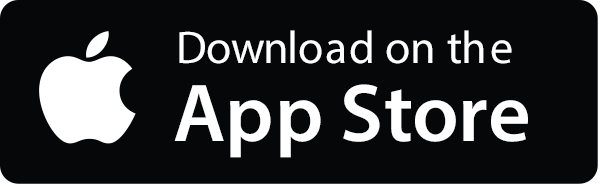
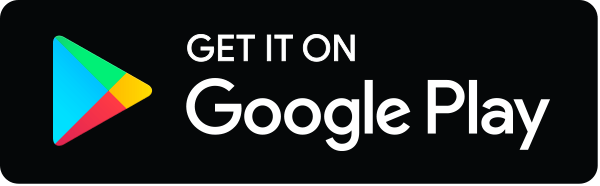