Fig. 20.1
Near-infrared (NIR) window tissue absorption coefficients. Between the wavelengths of approximately 700–900 nm, the oxygenated hemoglobin (OxyHb), deoxygenated hemoglobin (DeoxyHb), water, and lipid absorption spectra reach a minimum; this makes this window ideal for in vivo imaging. “Recreated with kind permission of [Elsevier] [38]”
20.3 Fluorescence Imaging Technologies in the Clinic: Advantages and Limitations
Fluorescence imaging is by far the most widely used technology for in vitro and in vivo micro-/macro-imaging research. Where preclinical whole-body NIR fluorescence imaging is extensively used, its main advantages include being easy to use, safe, low cost, and highly versatile. Nevertheless, NIR fluorescence imaging contrary to other modalities, such as ultrasound, CT, MRI, and PET, is still lagging behind widespread human use in the clinic. However, NIR fluorescence imaging has revealed growing potential in this field, recently reviewed [39–42].
Briefly, NIR fluorescence has been associated with fiber-optic endoscopy techniques, namely in the diagnosis of suspected lesions and assisted target intervention, in gastrointestinal [43] and lung cancer [44]. Endoscopy is already an established optical-based technology that only needs to be upgraded with the fluorescent component, which represents a major advantage for its clinical translation [41, 42].
Additionally, NIR fluorescence imaging using contrast probes can provide real-time image data for tumor guided resection [45], sentinel lymph node mapping [38, 40, 46], provide contrast to identify critical structures such as nerves [47] and blood vessels in reconstructive surgery [48]. Nevertheless, there are several technical aspects that require improvements for the above applications, namely for sentinel lymph node mapping or any open surgery application. NIR light is invisible to the human eye, so besides the excitation source and acquisition camera, the imaging systems need a display for the surgeon to visualize the fluorescent signal. Also, these procedures traditionally require the fluorescent acquisition to be performed in the dark; to minimize interference from the environment light, these factors may restricts surgical performance.
Recent studies proposed new imaging systems for the application of this technology on the surgical room [40]. These included the fluorescence-assisted resection and exploration (FLARE) a specialized intraoperative NIR fluorescence imaging system which easily allowed seeing the surgical anatomy with 3 image views: color, NIR fluorescence, and color-NIR merged [38, 49]. Furthermore, the integration of fluorescence imaging with minimally invasive robotic surgery, such as the da Vinci surgical system [50] could represent a powerful approach. Recently, this system was tested with the NIR dye (indocyanine green (ICG)) in a human study, to visualize the biliary anatomy during a cholecystectomy [51], identify renal vasculature, and differentiate renal tumors from normal tissue during a nephrectomy [52].
20.4 Semiconductor Quantum Dots
Semiconductor nanocrystal QDs have been one of most intensely studied fluorescent NPs for biomedical nanomedicine applications; especially in cancer research, extensive reviews are available [29, 53–58].
20.4.1 Quantum Dots Characteristics and Advantages
These nanocrystals present different light emission properties than traditional chemically synthesized dyes or fluorescent proteins. Briefly, the absorption of a photon with energy above the semiconductor bandgap energy results in the creation of an electron–hole pair (or exciton). The radiative decay of the exciton results in photon emission with wavelengths corresponding to the semiconductor’s bandgap. The absorption has an increased probability at higher energies (i.e., shorter wavelengths) and results in a broadband absorption spectrum [56].
The advantages of using QDs in the place of other traditional organic dyes have been widely reported [59, 60]. The unique optical properties of QDs include high absorbance, high quantum yield, low photobleaching, narrow emission bands, and large Stokes’ shifts. QDs are more chemically stable and are not so easily metabolized or degraded by the cells compared to fluorescent dyes. The emission spectra of QDs can be tuned across a wide range by changing the size and composition of the QD core, where the narrower emissions allow to resolve more wavelength peaks than possible with standard fluorescent dyes [61].
20.4.2 Quantum Dots Engineering Strategies for In Vivo Applications
QDs were first illustrated by Alivisatos et al. [62], and their potential for ultra-sensitive biological detection was unraveled by Chan and Nie [63]. Since the first reports describing this type of materials, engineering strategies have been employed to improve and tailor QDs physical, chemical, and optical properties to enable then to be used in biological systems. These include development of the size and chemical composition of the QDs core, shell, and hydrophilic coating structure, including conjugation with biomolecular ligands [29, 64].
Today, term “QDs” can be applied to a wide range of nanoparticle types with different compositions, but typically semiconductor QDs have a core/shell structure of 2–8 nm in diameter; normally, the core is composed of heavy metal semiconductor crystalline compounds such as cadmium selenide (CdSe), cadmium telluride (CdTe), indium arsenide (InAs), or lead selenide (PbSe) and a shell made of zinc sulfide (ZnS). The introduction of a shell made of a larger bandgap material such as ZnS was a major design advance. The shell confines the exciton to the core and passivates dangling bonds. This greatly enhances the QD, improves photostability, and provides resistance against environmental photooxidation [7]. Additionally, the bare QDs are not soluble in water or biocompatible as such, so another important design development that enabled their use in biological systems was the coating with a hydrophilic layer that ensured compatibility with physiological media and provided extra protection for the core [29, 56, 65]. Several strategies and different types of hydrophilic coating molecules have been described in the literature [7, 29, 64]. One of the most extensively used is the coating with PEG or other polymers; besides water solubility, it greatly reduced nonspecific cellular uptake of QDs [66–68]. The QDs surface was also functionalized with targeting biomolecular ligands, such as antibodies, peptides, or small molecules, used to target specific cells/tissues, and importantly tumor sites, NPs tumors targeting strategies have been recently reviewed [69–71].
20.4.3 Quantum Dots Toxicity In Vivo
The QDs main drawbacks are related to biocompatibility, clearance, and long-term toxicity issues. QDs injected intravenously in mice even when targeted to tumor accumulate in the body. They have been detected in the kidney, liver, lymph nodes, and bone marrow [72, 73], in some cases up to two years after injection, with a blue shift of the emission spectra due to NPs degradation [23]. The extent of degradation of the shell/coating is critical for toxicity, since they shield the release of highly toxic Cd from the core. The release of free Cd and other heavy metals compounds is one of the main safety issues raised regarding to use of QDs in humans [22].
Also, QD are highly reactive and are subject to photooxidation and air oxidation, with the formation of free radicals, another mechanism of toxicity [74]. The QDs toxicity issues have been extensively reviewed elsewhere [9, 75, 76]. Several engineering strategies to try to minimize this aspect have been described; some examples include the use of Cd-free QDs [77, 78]. Some works studied developments to maximize integrity on the shell/coating [30–32], and this should be effective only as long as the coating remains intact. Other strategies focus on enhancing clearance from the body, and low-sized QDs functionalized with tumor-specific small molecular ligands were tested in a mice model and shown to be cleared from the kidneys if the hydrodynamic diameter was less than 5.5 nm [79].
20.4.4 Quantum Dots Preclinical In Vivo Applications
The potential of QDs for preclinical tumor imaging and detection, including theranostics applications, has been recently reviewed [53, 54, 73, 80]. Examples of relevant studies include the following.
20.4.4.1 Sentinel Lymph Node Mapping
This was performed with NIR QDs in different types of animal tumors models, such as bladder [81], breast [82], esophageal [83], gastrointestinal [84], and lung [38, 85]. Relatively to traditional techniques (e.g., fluorescent dyes), QDs due to their high quantum yield present greater emission light penetration through thick tissues and superior signal to noise, minimizing the interference of autofluorescence tissue background [86]. In addition, for this technique size maters, as injections in the tumor of small NPs (<5 nm) can pass through multiple lymph nodes giving up false positives, QDs offer the possibility for precise engineered size to enable optimal location on the sentinel lymph nodes [86].
20.4.4.2 Primary Tumors Guided Surgery
20.4.4.3 Theranostics
QDs have been engineered to be also nanocarriers of therapeutics, combining drug delivery with imaging for monitoring the pharmacodynamics of the drugs and the effectiveness of the treatment. For example, a recent study described a pH-responsive QD–mucin1 aptamer–doxorubicin conjugate tested on an ovarian tumor mice model. Mucin1 represented the tumor-targeting ligand and doxorubicin was attached to the QD, quenching the fluorescent signal, through a pH-sensitive hydrazone bond. Inside the cells, the acidic environment promoted the release of the drug with the subsequent recovery of the fluorescent signal that could be detected [91]. Other studies employed QDs to track siRNA delivery and interference [92–96], although these were only tested in vitro with cultured cells, but reveal some potential for in vivo use.
Overall, despite the notable number of preclinical research performed with QDs and their high potential for biomedical clinical applications, there are no records of any intended or performed human clinical trials. Safety concerns related to their in vivo behavior represent by far the major limitation for their clinical use.
20.5 Fluorescent Dyes: Indocyanine Green Clinical Applications and Advantages of Dye-Loaded Nanoparticles
As described previously for in vivo imaging, the NIR window (~700–900 nm) of the spectrum provides higher penetration and lower host tissue interference with the light. For preclinical research, there are a vast selection of NIR organic dyes available [97–99] and other types of molecules (e.g., fluorescent proteins) [98]. Some examples include cyanine-based lipophilic dyes such as DiD (ex.644 nm; em.665) [100], DiR (ex.750 nm; em.782 nm) [101], hydrophilic dyes such as Cy5 (ex.650 nm; em.670 nm), Cy7 (ex.743 nm; em.767 nm) [102], and far-red engineered proteins such as the eqFP650 (ex.592 nm; em.650 nm) [103].
20.5.1 Indocyanine Green and other Clinically Relevant Dyes
Relatively to clinical applications very few dyes have been employed in human studies. Indocyanine green (ICG) is a 775-Da (Fig. 20.2) cyanine derivative dye (ex.760–785 nm; em.820–840 nm), first FDA approved in 1958 for indicator dilution studies [40].
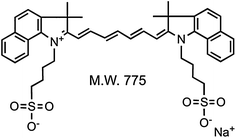
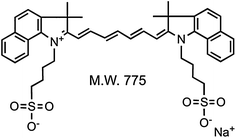
Fig. 20.2
Indocyanine green (ICG) molecular structure. “Recreated with kind permission of [Elsevier] [38]”
Today, ICG is still the only NIR dye approved by the FDA for human clinical use. One of its major applications is in sentinel lymph node mapping for image guided surgery following injection at the tumor site [40, 46]. ICG has also been used in reconstructive surgery, ophthalmic angiography, to evaluate cardiac output, hepatic function, and liver blood flow [40, 48, 104]. When injected intravenously ICG presents a very short lifetime in the blood, with a half-life of approximately 3–4 min [105]. ICG main issues are its weak fluorescent properties and low stability compared to other NIR dyes employed in preclinical studies [104]. Other clinical significant dyes include methylene blue [39, 106] and omocianine [107, 108], although these two were only approved to be use in selected clinical trials.
20.5.2 Advantages of Dye-Loaded Nanoparticles
Direct application of ICG or any other type of low molecular weight dyes in vivo can be limited by several factors. These include rapid clearance from the body and relative fast degradation of the dye with the correspondent loss of the fluorescent signal.
The use of engineered nanoplatforms where the dye is encapsulated or attached on the surface of the NPs represents an interesting strategy. This approach presents several advantages for tumor studies. These include: first, a versatile NP of controlled size and structure where is possible to include different probes for multimodal imaging and/or sensing, with the option to also include therapeutic agents [9–12]. Second, specify coating of the NPs is to increase the body circulation time, enhancing the passive accumulation in the tumor site and with the option to decorate the NPs external coating with tumor/tissue targeting ligands. Third, the association with the NPs material stabilizes and decreases the dye biological degradation. Fourth, because the dye is concentrated in the particle, there is an increase in brightness; although to high concentrations, it may produce the opposite effect due to self-quenching.
There are a number of different compounds and strategies described in the literature to produce NPs with incorporated dyes or other fluorescent components [3, 10, 109–113]. In the next sections, we will describe some of those subdivided into inorganic materials (calcium phosphate and silica) and organic complexes (polymeric and liposome based) (Fig. 20.3).
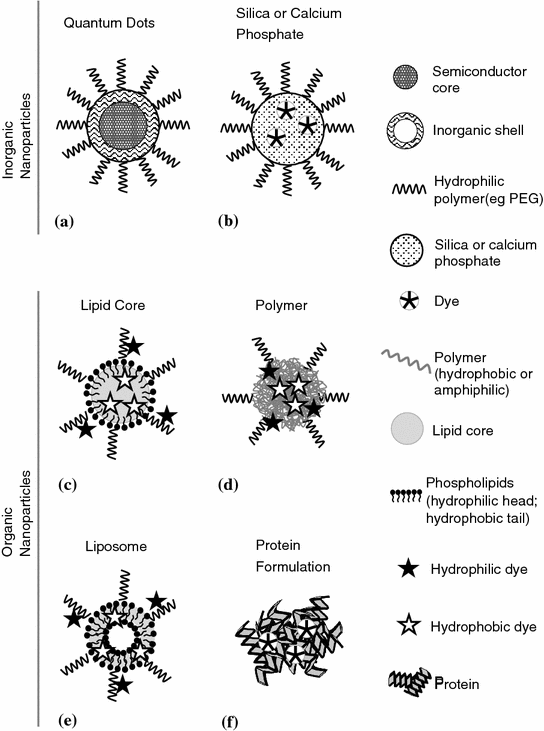
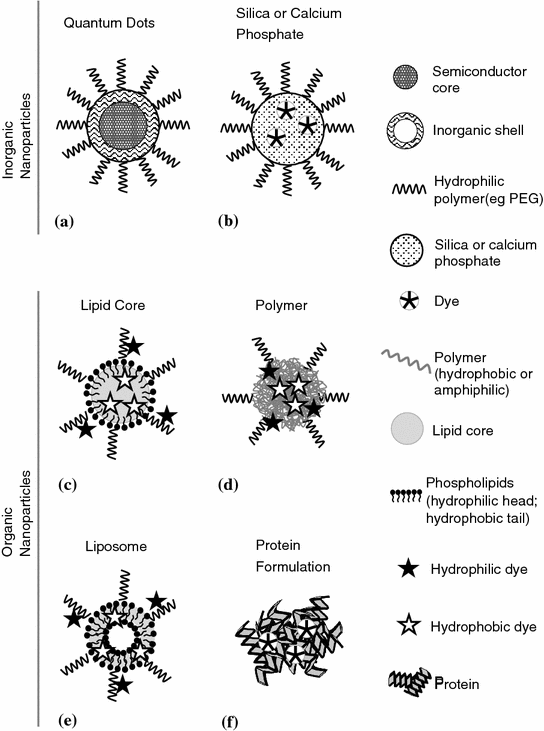
Fig. 20.3
Schematic representation depicting some types of fluorescent nanoparticles designs. Inorganic nanoparticles: a semiconductor quantum dots and b calcium phosphate or silica matrix loaded with organic dye and coated with a hydrophilic polymer. Organic nanoparticles loaded with dye, lipid or polymer based, with c lipid core, e liposome, d polymer self-assemble architectures, and f protein dye absorbed nanoparticles. Hydrophobic organic dyes can be loaded in the lipid core (c) or the phospholipid bilayer “shell” (e) and also within the polymer hydrophobic “core” groups (d), while hydrophilic organic dye can be conjugated to the polymers backbone (c, d, e). “Adapted with kind permission from [MDPI] [109]”
20.6 Inorganic Nanoparticles Loaded with Dyes
Generally, these NPs are composed of fluorescent dyes incorporated on an inorganic matrix (Fig. 20.3b). Several types of material can be included in these categories; many times, theses are multimodal NPs where beside the dyes, other types of materials (e.g., gold, iron oxide, gadolinium, and carbon particles) can be incorporated or linked to the inorganic matrix [114–118]. For the purpose of the present section, we will discuss two prevalent types of fluorescent inorganic NPs, calcium phosphate, and silica.
20.6.1 Calcium Phosphate Fluorescent Nanoparticles
Calcium phosphate is one of the most widely known agents for in vitro nonviral DNA/RNA delivery [119–121]. The precipitate NPs display a pH-dependent solubility profile and relatively low toxicity, are highly biocompatible and biodegradable, and therefore have been studied for imaging and therapeutic application with encapsulated oligonucleotides, but also drugs and dyes [122].
An important drawback for in vivo use is that calcium phosphate particles are unstable and present variable size, but engineering design refinements in the synthesis protocols and polymer coating (e.g., PEG) helped stabilize the structure [123, 124].
The potential for application in small animals imaging have been described using ICG-loaded calcium phosphate [125–127]. Despite the translational potential, relatively low toxicity and the fact that some injectable derivate products are already FDA approved for medical use (e.g., bone synthetic cement graph) [128], so far there are no records of calcium phosphate nanoparticle-based clinical trials.
20.6.2 Silica Fluorescent Nanoparticles
20.6.2.1 Characteristics and Advantages
20.6.2.2 Engineering Strategies for In Vivo Application
Silica NPs represent a versatile nanoplatform where fluorescent dyes and other functional molecules can be loaded inside the pores or covalent attached to the particles [130]. Importantly, the loaded dyes fluorescence properties remain quite stable in the silica matrix [34, 131–133], and this represents a major advantage for in vivo applications. Considering that these particles normally do not contain heavy metal components, in contrast to QDs, toxicity of silica NPs in the body is normally associated with mechanical vasculature obstruction of the organs that leads to blocking and organ failure [134]. It was demonstrated that both silica toxicity and biodistribution are influenced significantly by NPs porosity and surface characteristics, and the capacity for particles being able to be degraded and excreted out of the body by urinary and hepatobiliary routes [129].
Recent works described an interesting engineering strategy applied to fluorescence porous silica NPs loaded with anticancer drug doxorubicin, these could self-destruct in vivo into smaller components releasing the drug and be cleared out of the body within a relatively short period of time reducing the risk of causing damage to normal organs [24, 25].
20.6.2.3 Preclinical In Vivo Applications and Human Clinical Trials
There are a number of biological studies, recently reviewed [113, 130, 135, 136], performed with these types of particles, where silica NPs were successfully employed for small animal drug/gene delivery and imaging [114, 115, 137–141], commonly using a multimodal strategies [114–116, 118].
Most significantly, the so-called silica C-dots [133, 142] represent one of the first times an inorganic particle was approved to be used as a platform for both diagnostics and staging in advanced melanoma tumors in human clinical trials [143]. The C-dots were labeled with a radioactive probe and injected in the tumor site for PET detection of the surrounding lymph nodes. Although in the previous study the probe used was radioactive iodine, animal studies with Cy5-loaded C-dots also demonstrated the advantages of using NIR imaging for the localization of superficial lymph nodes [5]. This opens up the possibility for in the future being able to use already FDA approved NIR fluorescent dyes with these NPs. For example, other silica NPs loaded with ICG have already being described in several animal imaging studies [116, 117, 141, 144].
20.7 Organic Nanoparticles Loaded with Dyes
In these types of NPs, the dye is incorporated by either just adsorption or through a covalent bond to an organic structure; an extensive range of different organic materials and designs have been described [4, 145]. Also similar to the inorganic NPs, many times, these particles can incorporate other materials for multimodal imaging (e.g., PET, MRI) and/or a therapeutic component [4, 146, 147].
Organic NPs in general demonstrated effective properties as an in vivo nanocarrier, giving rise to several clinical contract agent conjugates and drug nanoformulation used in humans. Making them the most successful group of NPs to be translated to the clinic, this aspect has been recently reviewed [110, 145]. Two examples are as follows: Doxil, the first FDA approved nanoformulation in 1995 for AIDS-related Kaposi’s syndrome, which resulted from the incorporation of doxorubicin, within liposomes [148], and Abraxane, approved in 2005, is based on an albumin-bound formulation with paclitaxel, developed to treat metastatic breast cancer [149].
20.7.1 General Advantages and Issues
The main advantages of organic-based NPs include the capacity to enhance drugs efficiency without the need to alter the drug compound, improvement in the access to the target tissue/cells due to higher biological barriers crossing capacity, and importantly, they have proved to be safe for human use [4, 145]. This is because these particles are constituted by synthetic or natural occurring organic molecules, some of those already present in the human body, making them highly biocompatible and biodegradable.
The main issues are related to the organic NPs heterogeneity, namely in obtaining and maintaining reproducible NPs proprieties such as size, drug/dye load, structural architecture, and also their stability during storage and under physiological conditions. They normally are classified based on their composition/structure; we will briefly discuss two examples: lipid- and polymeric-based NPs.
20.7.2 Lipid Fluorescent Nanoparticles
20.7.2.1 Characteristics, Advantages, and Issues
Lipid NPs result from ensembles of lipophilic and/or amphiphilic lipids in small artificial vesicles of spherical shape (Fig. 20.3e and c), with a general diameter 50–300 nm [150, 151]. Different compositions and structures of lipid NPs have been described for imaging and therapy, such as nanoemulsions [152], nanocapsules [153, 154], solid lipid NPs [155], and liposomes [151, 156]. Due to their nature, they can for example be used to incorporate negative charged nucleic acid through electrostatic interactions with the positive charge phospholipids [156] and different lipophilic cargo, where drugs represent a major application [145].
The main issue of this type of particles is related to their colloidal stability; especially on living systems, they are relatively less stable than for example polymeric NPs [151].
20.7.2.2 Engineering Strategies for In Vivo Application
There are different strategies to improve stability, and this includes conjugation with polymeric moieties, such as PEG [147]. Another example involved the use of a complex mixture of different types of lipids (long-chain mono-, di-, and triglycerides) and other surfactants (phospholipids and PEG-stearate) to stabilize the NPs [152]. An importantly feature of lipid NPs is the option to encapsulate different of dyes such as DiD, DiR [153, 154, 157], and ICG [105, 158, 159] to obtain bright fluorescent NPs.
20.7.2.3 Preclinical In Vivo Applications
The engineering strategies used to improve the general particle stability also contribute to improving their fluorescence properties and decreasing the dye degradation. For example, in the case of nanoemulsions or nanocapsules, lipophilic dyes can be homogenously incorporated in the lipid core in high concentration (Fig. 20.3c) providing excellent brightness [150, 153]. Self-quenching phenomena, due to high dye loading, have to be monitored, and dye leakage can be a greater issue on the lipid NPs, compared to more stable dye-loaded inorganic or polymeric NPs [33]. Nevertheless, they were some recent studies with lipid NPs, termed Lipidots, composed of DiD-loaded lipid core that claimed comparable results to commercial QDs with much lower toxicity, when used as a fluorescent contract agent for lymph node mapping in mice [109, 150]. Other preclinical studies on small animal have been performed to demonstrate the application of ICG-loaded lipid NPs for sentinel lymph node mapping [160] and general tumor imaging [161, 162]. Despite some lipid nanoformulations already in use in the clinic [145, 148], there are no records of human approved fluorescent lipid NPs.
20.7.3 Polymeric Fluorescent Nanoparticles
20.7.3.1 Characteristics and Advantages
Polymeric NPs present a variety of structural arrangements and are formulated with different polymer constituents. The different structures are related to the self-assembly of the hydrophobic/hydrophilic polymer subunits and can include other compounds, such as dyes [109] and therapeutic drugs, extensively reviewed elsewhere [111, 145]. Relevant examples of NPs architectures have been described, and this included polymeric micelles [163], dendrimers [164], and polymer capsules [165]. Some examples of prominent synthetic polymer constituents used on the design of these NPs are PEG, poly(lactic acid) (PLA), poly(D,L-lactic-co-glycolide) (PLGA), and poly(caprolactone) (PCL). Other important described constituents are natural occurring polymers such as poly(glutamic acid) (PGA), poly(lysine), saccharides (dextran and chitosan) and body present polymers (hyaluronic acid and human serum albumin) [109, 145].
The common advantages of these polymers are their high biocompatibility and/or biodegradable proprieties, plus being relatively safe. Several studies described polymer-based NPs used as nonviral gene delivery system [166–168], but the vast majority of works with these NPs are related to their use as a nanocarrier for therapeutic drug delivery [111, 165, 169] where these particles presented greater clinical success [145]. It is also common for these particles to be loaded or conjugated with fluorescent dyes for imaging [110] or distinct theranostic applications [170].
20.7.3.2 Engineering Strategies for In Vivo Application
Some of main advantages and associated engineering rational in the development of these NPs are related to the increase in drug solubility and in turn bioavailability, controlled delivery with accumulation on target locations, maintenance of systemic drug concentration (i.e., increasing serum half-life) and demonstrated safety for human used [111, 145]. For example, PEG is one of the most widely used polymers to increase circulation time on NPs and has been approved for human use [169]. Similar to the previously described lipid NPs, different polymer compositions/designs provide efficient loading and delivery of the drugs or/and fluorescent dyes, enable degradation and excretion from the body, but importantly should reveal lower toxicity [171].
Polymer NPs are high versatile allowing for different designs and the conjugation with diverse materials (e.g., gold, iron oxide, carbon particles) resulting in multifunctional/multimodal NPs [4, 10, 146, 147].
Highly relevant for optical imaging is the incorporation of fluorescent dyes. Different approaches have been described; for example, NIR lipophilic dyes such as DiD and DiR [172] can be incorporated on the polymer hydrophobic “core.” While hydrophilic dyes, such as Cy5.5, can be covalently conjugated to the polymers backbone (Fig. 20.3d), this improved stability and reduced dye leaking from the NPs [170, 173, 174]. Hyaluronic acid (HA) is a polysaccharide present in the extracellular matrix and synovial fluids of the body, making it extremely biocompatibility and biodegradable [175]. HA-based self-assemble NPs represent an interesting versatile platform where is possible to load hydrophobic drugs/dyes in the hydrophobic “cores” or covalently attach them to the polymer backbone. Furthermore, they revealed specific targeting to tumor sites, due to strong receptor-binding affinity to CD44, overexpressed in most tumor cells [176]. The covalently attached Cy5.5-dye-labeled HA NPs demonstrated to be relatively stable under physiological conditions and presented good fluorescence properties. They have been successfully used for tumor imaging [177–179] and theranostic applications [170, 180].
20.7.3.3 Preclinical In Vivo Applications and Human Clinical Trials
For example, recently HA-based NPs were engineered as a theranostic system combining early tumor detection with targeted tumor therapy and evaluated on a colon cancer mice model [170]. The diagnostic consisted of Cy5.5 chemically conjugated to the HA that successfully allowed fluorescence imaging detection of small-sized colon tumors as well as liver-implanted colon tumors on live mice. The target therapy part consisted of the anticancer drug irinotecan encapsulated into the hydrophobic HA cores, that was effective on suppressing tumor growth, and this response was also possible to be monitored through fluorescence imaging [170].
Several researchers used the clinically significant ICG dye to perform preclinical studies. The dye was encapsulated on polymeric NPs such as PLGA [181, 182] and PEG-folate-conjugated ring-opening metathesis polymerization-based copolymers [183] for mice tumor imaging. Concretely sentinel lymph node mapping was demonstrated with ICG-loaded PGA [184] and HA NPs [185, 186].
Importantly, ICG has been noncovalently absorbed/complexed with human serum albumin in a NP formulation (Fig. 20.3f), revealing a hydrodynamic diameter of around 7 nm, which improved the optical properties, resulting in quantum yield enhancement, compared to free ICG [187, 188]. Most significantly, both ICG individually and albumin-related drug complexes, such as Abraxane [149], are already approved for human use. This may help explain why albumin–ICG was one of only polymeric fluorescent NPs approved for human clinical trials so far [40].
Invasive tumors spread from their original site through the lymph channels to regional nodes, and the injection of a contrast agent at the tumor site allows the surgeon to easily identify and take biopsy of the first or sentinel lymph nodes which the cancer encounters. If the analysis of the sentinel lymph node reveals that is free of tumor cells there is high probability all the lymph nodes in the region are also free, this spares the patient from unnecessary greater surgical procedure to remove all the lymph nodes. Albumin–ICG NPs were used on human clinical trials for sentinel lymph node mapping in breast [189], cervical [190], head and neck cancer [191], and vulvar [192] (Fig. 20.4) cancers. All these human trials were performed using the mini-FLARE imaging system [49]. But the da Vinci minimally invasive robotic surgery system was used recently with ICG to image human biliary anatomy [193], and there are ongoing clinical trials for endometrial cancer sentinel node mapping [194] and to help identify renal cortical tumors [195].
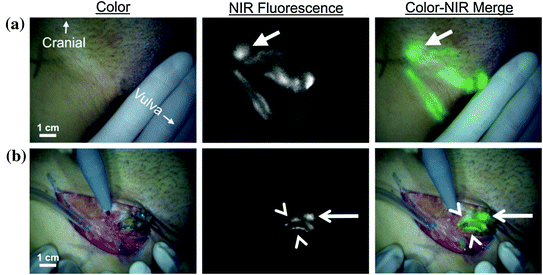
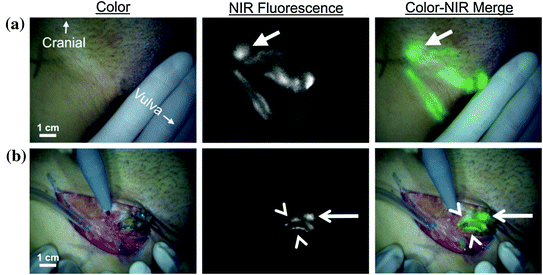
Fig. 20.4
Sentinel lymph node (SNL) mapping using near-infrared (NIR) fluorescence imaging FLARE system in a human patient with vulvar cancer. a Peritumoral injection of 1.6 mL of 500 μM indocyanine green human serum albumin (ICG:HAS), injection site covered by hand, identifies lymphatic channels, which converge in a SLN (arrow) that can be seen percutaneously (top row). b Identification of the SLN (arrow) and 2 afferent lymphatic channels (arrowheads) is demonstrated using NIR fluorescence imaging 17 min after injection of ICG:HSA (bottom row). Scale bars represent 1 cm. “Recreated with kind permission of [Elsevier] [192]”
20.8 Conclusion and Future Perspectives
20.8.1 Fluorescence Imaging Technologies in the Clinic
Despite fluorescence imaging widespread use in preclinical research with NPs and other probes, this has not yet translated to the clinic, where others technologies (i.e., ultrasound, CT, MRI, and PET) are already well established. Nevertheless, there is a growing potential in this field [39–42].
For example, fluorescent endoscopy represents a potential application without the need for major technological adaptation [41, 42], and other fluorescence technologies, such as intraoperative imaging systems, are being evaluated for the translation to the surgical environment [48].
In our perspective, we believe that specialized intraoperative NIR fluorescence imaging systems, such as the FLARE [38, 49], and more recently, minimally invasive robotic surgery [50] technologies represent important developments that could potentiate the use of fluorescent NPs in the clinic. Minimally invasive robotic surgery systems combined with fluorescence imaging are especially interesting, as the surgeon performs all the procedure through a display video system, they can more easily engage surgery on both color and fluorescence modes, providing an excellent interplay between the “normal” tissue color view and fluorescence imaging. Although this system at this moment is highly specialized and not common, this could contribute to bringing fluorescence imaging to routine use in the clinic.
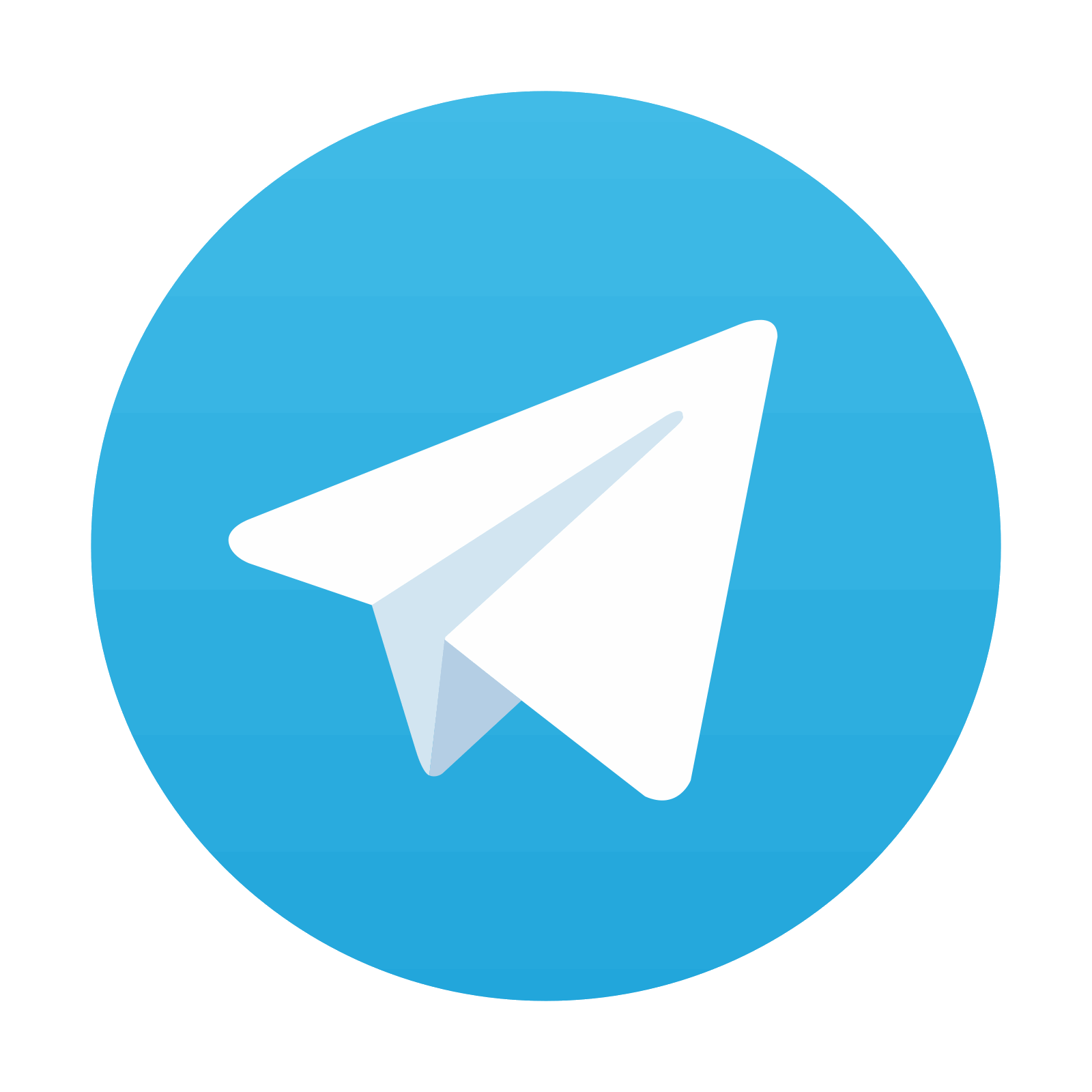
Stay updated, free articles. Join our Telegram channel
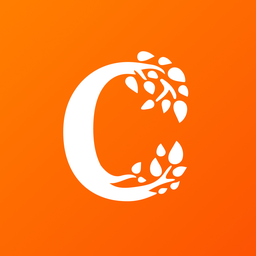
Full access? Get Clinical Tree
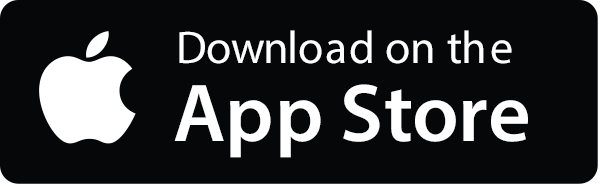
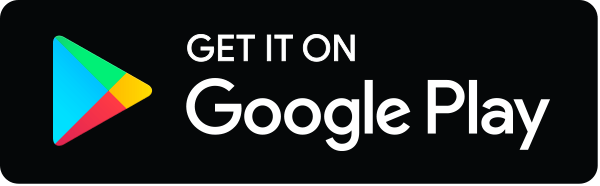