Engineered nanostructure
Stem cell
Differentiated cell
Proposed mechanism
Reference
LBL-assembled SWCNT/PEI polyelectrolyte composite
mNSCs
Neurons, oligodendrocytes, astrocytes
Chemical structure better suitable as neural electrode
[36]
LBL-assembled SWCNT/laminin composite
mNSCs
Neurons
Chemical structure better suitable as neural electrode
[37]
MWCNT micropatterns network
hNSCs
Neurons and astroglial cells
Nanotopography
[38]
Monolayer of SWCNT micropatterns network
hMSCs
No differentiation
[39]
Monolayer of SWCNT micropatterns network modified by fibronectin
hMSCs
No differentiation
[40]
SWCNT film
hMSCs
Neurons
Nano roughness
[41]
MWCNT sheet
hMSCs
Neurons
Nano roughness
[42]
SWCNTs/collagen matrix rigid fibril bundles
hESCs
Ectodermal lineage
Preconcentration platform
[43]
MWCNTs (PAA/PMA) thin film
hESCs
Neuron
Preconcentration platform
MWCNTs/PEG thin film
hMSCs
Osteocytes
Nanotopography
[47]
MWCNT/PCL composite
BMSCs
Osteocytes
Nanotopography
[48]
SWCNT monolayer
hMSCs
Osteocytes
Nanotopography
[49]
Aligned CNT network
hMSCs
Osteocytes
High cytoskeletal tension
[50]
3D CNT rope
hNSCs
Neurons
Electrical stimulation
[51]
3D porous US-SWCNTs/PPF composite
Rat MSCs
No differentiation/in vivo implantation
MWCNTs/PLA
hMSCs
Cardiomyocytes
Electrical stimulation
24.3.1 Carbon-Nanotube-Based 2D Scaffold for Tissue Engineering
The successful growth and differentiation of stem cells on CNT-based 2D scaffold was initially demonstrated by Jan et al., who studied the behavior of mouse cortical neural stem cells (NSCs) on layer-by-layer (LBL)-assembled SWCNTs [36]. The NSCs grown on this SWCNT/polyethyleneimine (PEI) polyelectrolyte composite multilayer thin film showed increased differentiation into neurons and oligodendrocytes and decreased differentiation into astrocytes compared to NSCs grown on poly-l-ornithine (PLO), one of the most widely used substrata for neural stem cells.
Similarly, SWCNT/laminin composite fabricated by LBL method was also reportedly found to support growth, proliferation, and differentiation of NSCs into neurons [37]. Extensive functional neural networks along with the presence of synaptic connections were observed on this composite scaffold. Furthermore, application of pulsed electrical stimulation through the SWCNT substrate revealed generation of action potential with simultaneous excitation of NSCs. The above results indicate the potential application of these SWCNT composite materials for neural tissue engineering and nerve injury repair using neural stem cells due to their chemical structure better suitable as neural electrode leading to their long-term integration with neural tissues.
Behavior of hNSCs and their selective neuronal differentiation has also been investigated on different MWCNT network patterns [38]. Topographical cues obtained from these micropatterns were found to provide optimal surface roughness for selective growth and adhesion of hNSCs on to MWCNT regions. Furthermore, differentiation of these hNSCs into neurons or astroglial cells was found to depend on shape and dimensions of the MWCNT micropatterns. The results suggest that these CNT patterns were able to induce the adhesion, growth, and selective differentiation of hNSCs even better than conventional cell culture substrates such as bare glass.
CNT micropatterns based on SWCNTs have also been reported for growth and morphogenesis of human mesenchymal stem cells (hMSCs). These micropatterns were fabricated by adsorbing SWCNTs onto self-assembled monolayer patterns and further modified with adhesion protein such as fibronectin [39, 40]. The hMSCs growing on these patterns exhibited a stronger affinity to SWCNTs region with an elongated morphology compared to bare glass, CNT, or only fibronectin regions. Further studies have reported upregulation of neurogenic expression of hMSCs grown on SWCNT film [41] and aligned MWCNT sheets [42]. hMSCs showed increased neurite outgrowth along the CNTs implying that the differentiation of hMSCs is controlled by the nanoroughness of the CNTs. The above results provide solid evidence that CNT-based nanofibrillar surfaces mimic the characteristics of ECM with enhanced cell adhesion and growth factor adsorption and promote stem cell differentiation. In other words, the role of ECM molecules in cell–nanostructure interactions is important in designing nanostructure-based surface arrays to control and monitor activity and behavior of stem cells.
CNTs modified with natural or synthetic polymers have also been reported for growth and differentiation of pluripotent cells like human embryonic stem cells (hESCs). The hESCs grown on SWCNTs grafted into collagen matrix were found to form rigid fibril bundles with polarized growth [43]. Additionally, 90 % of the hESCs were differentiated into ectodermal lineage indicated by their long and bipolar filaments aligned with CNT/collagen matrix. Similarly, hESCs grown on MWCNTs grafted with poly(acrylic acid) [44] (PAA) and poly(methacrylic acid) [45] (PMA) via in situ polymerization showed not only better viability but also enhanced differentiation into B-tubulin-positive neurons compared to thin films made up of individual polymer or PLO. Further surface analysis and cell adhesion studies indicated that above result could be due to enhanced cell adhesion and protein adsorption resulting from large surface area and nanoscale fiber morphology created by CNTs. The lineage selection in hESCs generally depends on growth factors and small molecules in the media and the microenvironment provided by the scaffold. These results confirm the responsiveness of the hESCs to matrix properties and thereby provide a simple and efficient way to direct hESC differentiation. Specifically, in these cases, CNT/polymer composite showed potential for their future application in forming neural-cell-based biodevices.
Similar to the above results pertaining to use of CNT and its composite for neural tissue engineering, many researchers have also investigated the suitability of CNTs as a substrate material for bone tissue engineering. Initial in vivo investigation showed high bone–tissue compatibility, new bone integration, and accelerated bone formation stimulated by recombinant human bone morphogenetic protein-2 (rhBMP-2) along with little local inflammatory reaction for MWCNTs adjoining bone [46]. This result encouraged several studies exploring use of engineered carbon nanomaterials as scaffold for bone tissue engineering. One such study done by Nayak et al. explored the homogenous thin film formed by polyethylene glycol (PEG)-conjugated multiwalled carbon nanotubes sprayed on to plain coverslips to induce hMSCs differentiation into osteogenic lineages (Fig. 24.1) [47]. This compact and thin film of MWCNT–PEG having nanometric irregularities was able to provide suitable microenvironment for differentiation of hMSCs into osteogenic lineage visualized by upregulation of osteogenic proteins like osteocalcin and osteopontin, without the need of any biochemical inducers like BMP-2. This study clearly demonstrates that such carbon nanomaterial-based scaffolds can direct and support differentiation of multipotent stem cells like hMSCs into other tissue types through subtle modification of the matrix and induction with specific media.
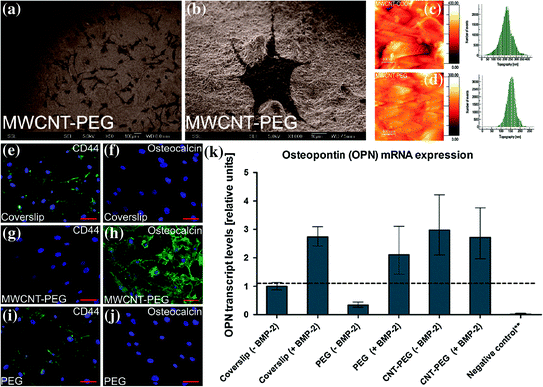
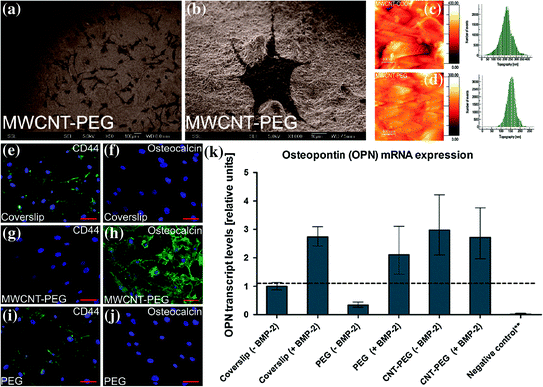
Fig. 24.1
a, b SEM images of hMSCs growing in normal medium on MWCNT–PEG-coated coverslips at day four of incubation showing growth of lots of cells in a large field of view (Scale bar is 100 μm) and growth of a single cell in a small field of view (Scale bar is 10 μm), respectively. c, d Atomic force microscopy images showing the topography of the surfaces of MWCNT–COOH- and MWCNT–PEG-coated coverslips, respectively. e–j Immunofluorescence image of cells subjected to osteoinduction without BMP-2 (Scale bars are 100 μm). e, f Plain coverslips showing the presence of CD44 and absence of OCN; g, h MWCNT–PEG-coated coverslips showing the absence of CD44 and presence of OCN; i, j only PEG-coated coverslips showing the presence of CD44 and absence of OCN. k qPCR analysis of relative expression levels of OPN for hMSCs cultured on different types of substrates and osteoinduced with osteogenic media with or without BMP-2 for 14 days. **Negative control consists of a coverslip without BMP-2 and without induction with osteogenic media. Figure adapted from Nayak et al. [47]. Copyright@ACS
Similar CNT/polymer composite scaffolds based on MWCNTs/polycaprolactone (PCL) were fabricated by the solution evaporation technique and have been studied for attachment, proliferation, and differentiation of the rat bone marrow-derived stromal cells (BMSCs) [48]. Incorporation of MWCNTs into PCL led to increase in surface roughness and tensile strength of the matrix. The proliferation and osteogenic differentiation of BMSCs were found to depend on the concentration of MWCNTs in the polymer, and the optimum concentration of MWCNTs to grow BMSCs was 0.5 %.
The results from another study pertaining to growth of hMSCs on SWCNT monolayer showed enhanced adhesion, proliferation, and osteogenic differentiation due to oxygen plasma treatment without any induction medium [49]. The osteogenic proteins such as osteocalcin, alkaline phosphatase (ALP), and early markers for osteogenic commitment such as CBFA1 were found to be upregulated. The possible explanation could be stress on hMSCs due to enhanced cell spreading brought about by chemical effects along with normal topological effects. In addition to the above effects, enhanced proliferation and osteogenic differentiation were also exhibited by hMSCs growing on aligned CNT networks compared to those on randomly oriented CNT networks [50]. In other words, hMSCs grown on CNT networks could recognize the arrangement of individual CNTs in the CNT networks, which implies that direction of growth and differentiation of hMSCs can be controlled by alignment direction of the individual CNTs. In this study, enhanced proliferation and osteogenic differentiation were proposed to be due to mechanotransduction pathways triggered by high cytoskeletal tension in the aligned hMSCs.
24.3.2 Carbon-Nanotube-Based 3D Scaffold for Tissue Engineering
CNTs have been engineered to develop various 3D architectures, and several of these structures have been found to support growth, proliferation, and differentiation of NSCs, MSCs, and pluripotent hESCs. A 3D rope like structure with a diameter of 1 mm and length of 1.5 cm was prepared by elongating bulk CNTs and twisting the resulting CNT strips. This rope was evaluated as a scaffold for growth and differentiation of NSCs. NSCs growing on these CNT ropes displayed enhanced differentiation into neurons even in the early culture stage compared to conventional tissue culture plates [51]. In addition, the neurite outgrowth was found to polarize along the spiral direction of the rope. Furthermore, electrical stimulation led to increased neuronal maturity with simultaneous increase in speed of neurite outgrowth as determined by upregulation of specific protein markers such as B-tubulin class III and microtubule-associated protein 2 (MAP-2). The above synergistic results pertaining to enhanced differentiation into mature neurons and promotion of neurite outgrowth due to electrical stimulation indicates potential application of such electroconductive CNT rope for therapeutic application of neural tissue regeneration.
The first 3D porous biodegradable scaffold for bone tissue engineering was fabricated by reinforcing poly(propylene fumarate) (PPF) with ultra-short (US) SWCNTs. This porous US-SWCNT nanocomposite scaffold demonstrated good cell attachment and sustained proliferation for rat MSCs [52]. The in vivo biocompatibility of this scaffold was evaluated in a rabbit model by implanting them in femoral condyles and in subcutaneous pockets (Fig. 24.2) [53]. Addition of US-SWCNTs did not change the scaffold’s structure, but improved its mechanical properties. The porous composite scaffold exhibited favorable hard and soft tissue responses and a threefold greater bone tissue ingrowth compared to control polymer scaffolds as determined by microcomputed tomography (microCT), histology, and histomorphometry at 4 and 12 weeks after implantation. The results indicate that presence of US-SWCNTs may be assisting in osteogenesis by rendering nanocomposite scaffolds bioactive.
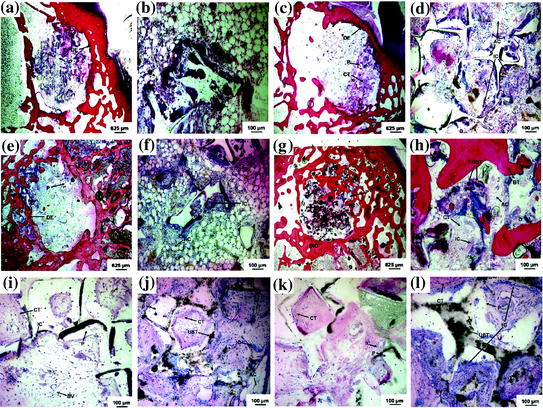
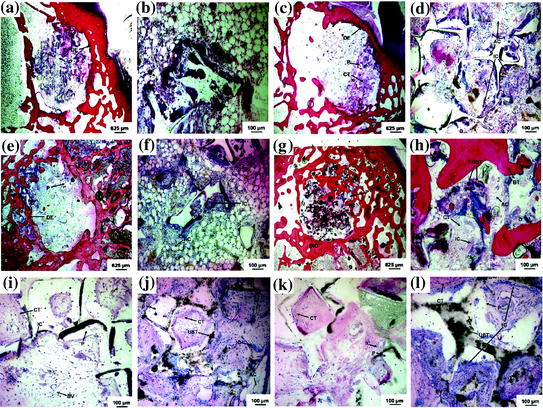
Fig. 24.2
Representative histological sections of CNT-based 3D scaffolds implanted in femoral condyle defects (a–h) and subcutaneously (i–l): a, b, and i a PPF scaffold 4 weeks after implantation, c, d, and j a US-tube/PPF scaffold after 4 weeks, e, f, and k a PPF scaffold after 12 weeks, and g, h, and l a US-tube/PPF scaffold after 12 weeks implantation. The PPF scaffold (P) appears in all images as white areas. The US-tubes (UST) appear as black aggregates in the nanocomposite images and are distinctly different from the other black artifacts generated during the sectioning process. Direct bone implant contact (BIC) occurred with the US-tube/PPF nanocomposite scaffold 12 weeks after implantation. The original defect edge (DE) is visible in the low magnification images. Connective tissue (CT), adipose cells (AC), blood vessels (BV), and inflammatory cells (IC) are also visible. Figure adapted from Sitharaman et al. [53]. Copyright@Elsevier
Another method of fabricating 3D porous biocompatible scaffold that could possibly mimic the structure of ECM is electrospinning which helps in easy incorporation of CNTs into polymeric nanofibers by spinning a homogenous mixture of CNTs and polymers. Scaffolds made up of such CNT composites have been found to possess enhanced tensile modulus. The behavior of such electrospun scaffold was initially evaluated by incorporating varying concentration of MWCNTs into poly(lactic acid) (PLA) and seeding adipose-derived mesenchymal stem cells on them [54]. At the end of two weeks, hMSCs were found to be alive and proliferating as determined by DNA quantification and live and dead cell assay. Moreover, hMSCs growing on the 1 % MWCNT composite showed morphological differences as they were closely packed and longitudinally aligned compared to hMSCs growing on PLA only scaffold. Recently, another study done on the MSCs cultured on SWCNT/PLA nanocomposite and subjected to electrical stimulation showed upregulated expression of cardiac gene and proteins [55]. Several other studies have also been done utilizing these electrospun CNT composite scaffolds for growth and proliferation of cells such as osteoblasts [56] and skeletal myoblasts [57].
24.4 Graphene
Graphene is a 2D sheet of sp2 bonded one-atom-thick monolayer of carbon atoms arranged in a honeycomb structure [58, 59]. It is a basic building block for other graphitic materials such as graphite, CNT, and fullerene. Because of their unique and desirable characteristics, graphene and its derivatives such as graphene foam, graphene oxide (GO), reduced graphene oxide (rGO), and GO nanocomposites have received extraordinary attention for a wide variety of applications such as nanoelectronics, sensors, and energy storage [60]. In addition, single-layered graphene has ultra-high surface area as every atom is exposed on its surface allowing efficient loading of drugs/genes leading to versatile surface functionalization. Therefore, within the realm of biomedical engineering, graphene-based nanomaterials are currently being explored as novel nanoplatforms for drug and gene delivery, multimodal imaging, image-guided cancer therapy including tissue engineering and regenerative medicine [60, 61]. While there is a continuous search for biocompatible scaffolds with desired physical, chemical, and mechanical characteristics for engineering appropriate biomimetic materials [47], graphene and its derivatives with unique properties such as high elasticity, flexibility, and adaptability to flat or irregular shaped surfaces can play key roles in promoting the adhesion, proliferation, and differentiation of various stem cells such as hMSCs, hNSCs, and iPSCs [62–64]. Table 24.2 lists scaffolds engineered from graphene and its derivatives for tissue engineering with various stem cells.
Table 24.2
List of graphene–based engineered scaffolds for tissue engineering with stem cells
Engineered nanostructure | Stem cell | Differentiated cell | Proposed mechanism | Reference |
---|---|---|---|---|
Graphene coated on different substrates (Glass, SiO2, PET, and PDMS) | hMSCs | Osteocytes | Preconcentration platform | [68] |
GO film with varying surface roughness | hMSCs | Osteocytes | Increased cytoskeletal tension due to nanotopography | [71] |
Graphene layer | hNSCs | Neuron | Good electrical coupling | [74] |
Fluorinated graphene | hMSCs | Neurons | Electrostatic induction resulting from polarization effect of carbon–fluorine bond | [75] |
GO coated on SiO2 | hMSCs | Adipocytes | Electrostatic interaction | [69] |
GO coated on SiO2 | iPSCs | Endodermal lineages | Physiochemical properties of surface | [76] |
Graphene foam | hNSCs | Neurons and astrocytes | Efficient conductive platform | [86] |
Graphene foam | hMSCs | Osteocytes | – | [87] |
24.4.1 Graphene-Based 2D Scaffold for Tissue Engineering
The potential of graphene as 2D biocompatible scaffold was initially evaluated as a substrate for mammalian NIH 3T3 fibroblast cells [65], mammalian colorectal adenocarcinoma HT-29 cells [66], human osteoblasts, and mesenchymal stromal cells [67]. It was found to promote growth, proliferation, and adhesion of these mammalian cells. Cellular functions such as gene transfection and expressions were also found to be enhanced on graphene-based scaffolds without any toxicity. In addition, mesenchymal stromal cells grown on graphene showed spindle-shaped morphology compared to normal polygonal-shaped morphology achieved on SiO2 substrate. While all of the above results suggested high affinity of mammalian cells toward scaffold based on graphene family of nanomaterials, the results pertaining to difference in morphology of mesenchymal stromal cells indicated its future application on regulating specific lineage direction of pluripotent or multipotent stem cells. Since then, several studies have been reported for differentiation of hMSCs, hNSCs, IPSCs, and hESCs growing on scaffolds based on graphene family of nanomaterials into specific lineages.
One such study was done for evaluation of graphene coated on different substrates (glass, SiO2, PET, and PDMS) for osteogenesis of hMSCs [68]. Graphene not only supported unhindered growth and proliferation of hMSCs irrespective of the below substrate but also accelerated their differentiation into osteocytes as indicated by enhanced expression of typical protein marker for osteoblasts such as osteocalcin, osteopontin, and calcium deposition (Fig. 24.3). The rate of osteogenic differentiation was found to be comparable to that of specific differentiation factor such as BMP-2. This particular result was further confirmed by Lee et al., who attributed the role of unique physiochemical properties of graphene surface which act as a preconcentration platform due to the noncovalent binding of growth factors including osteogenic inducers [69].
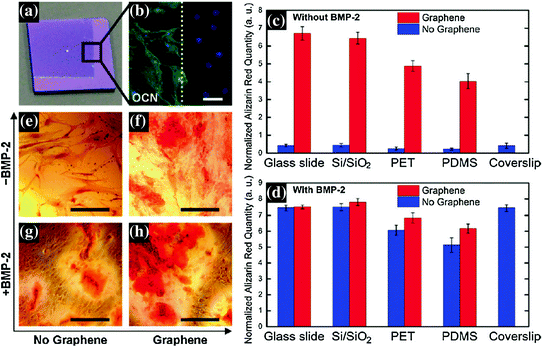
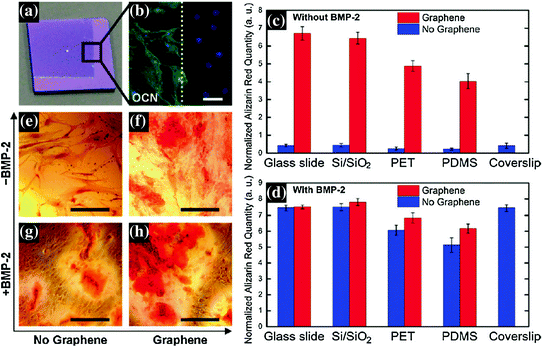
Fig. 24.3
a Optical image of 1 × 1 cm, partially graphene-coated Si/SiO2 chip, showing the graphene boundary. b Osteocalcin (OCN) marker showing bone cell formation on the same chip only on the graphene-coated area; the graphene boundary is also clearly visible here. c, d Alizarin red quantification of hMSCs grown for 15 days on substrates with or without graphene coating. c Cells grown in the absence of BMP-2. Control with coverslips is shown as a reference. d Cells grown in the presence of BMP-2. Conventional plain coverslips were used as a positive control. e–h PET substrate stained with alizarin red showing calcium deposits due to osteogenesis. e PET without BMP-2 and without graphene; f PET without BMP-2 and with graphene; g PET with BMP-2 and without graphene; h PET with both BMP-2 and graphene. Scale bars are 100 μm. Figure adapted from Nayak et al. [68]. Copyright@ACS
Since some of the prior studies have reported significant increase in osteogenic differentiation of hMSCs growing on scaffolds with nanotopography [70], therefore, surface morphology of graphene owing to its highly wrinkled structure has also been proposed to be the driving force for osteogenic differentiation [68, 69]. This role of surface topography was evaluated by growing hMSCs on graphene oxide (GO) films with different surface roughness created by crosslinking methacrylate functionalized GO by the process of free radical polymerization [71]. The extent of mineralization as determined by calcium precipitation on the roughest GO film was found to be four times higher than the smoother GO film. Moreover, spontaneous osteogenic differentiation of hMSCs was observed on rough GO film without the use of any chemical inducers. The above result was attributed to the increased cytoskeletal tension created due to the nanotopography on the surface of GO film which provides more anchoring points for the adhesion and hence facilitates the proliferation and differentiation of hMSCs. Such kind of engineering of graphene nanomaterials has opened up the possibility of preparing biocompatible scaffolds for potential use in bone tissue engineering and regenerative medicine.
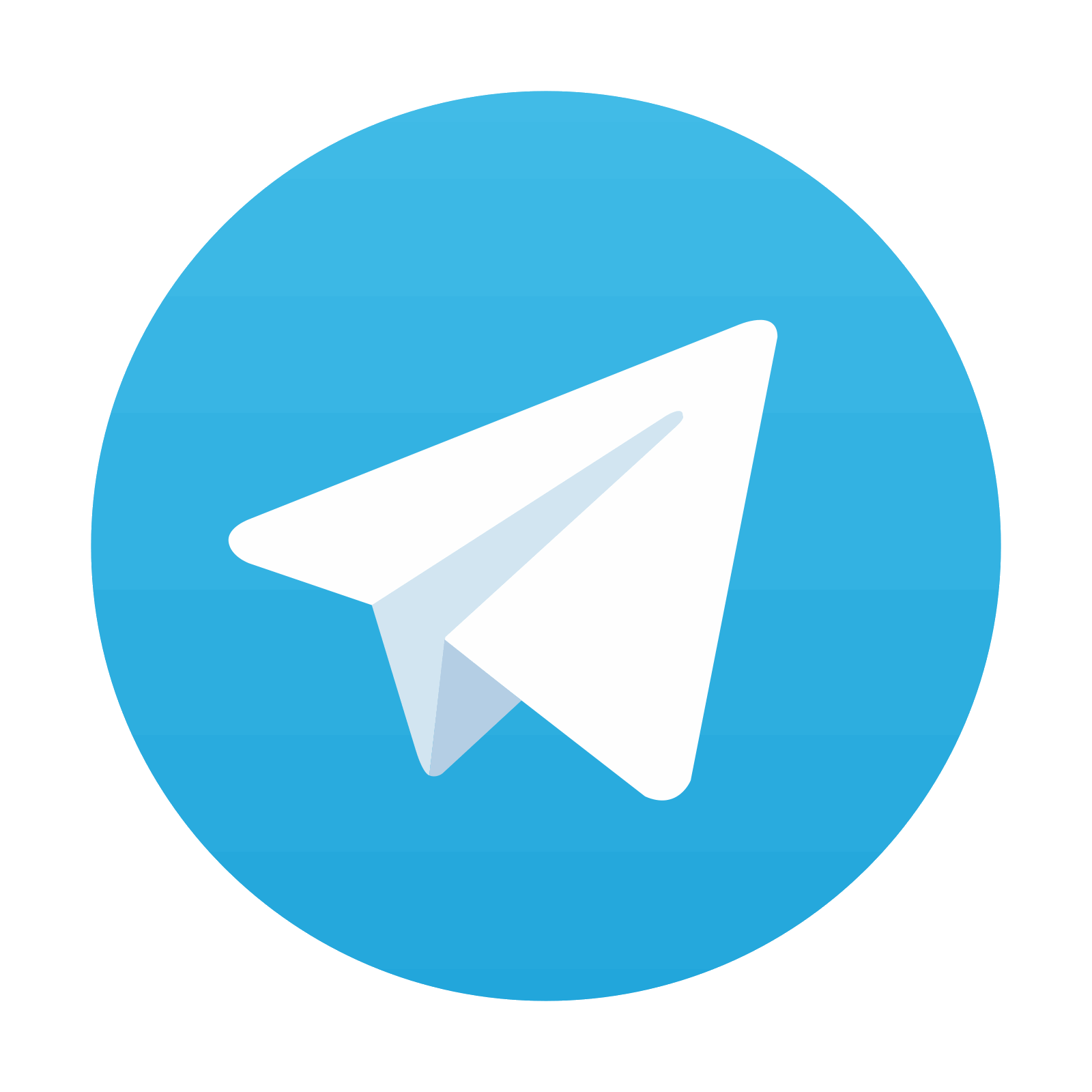
Stay updated, free articles. Join our Telegram channel
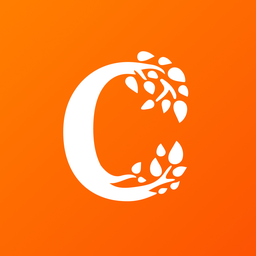
Full access? Get Clinical Tree
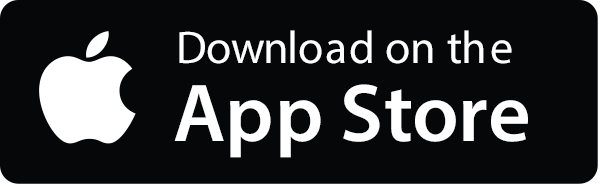
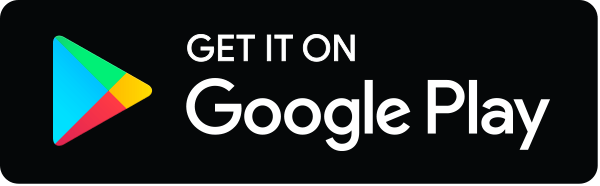