Fig. 15.1
The general procedure for systematic evolution of ligands by exponential enrichment (SELEX). Recreated with kind permission of Elsevier Ltd [100]
15.2.2 Improved SELEX
Along with the continuous development, a series of improving methods for SELEX have been developed, with the purpose of enhancing the efficiency of the selection or increasing the universality of aptamers [26, 27].
The most critical step of SELEX is to separate the binding sequences from the unbound ones, which can affect the quality of aptamers and the speed of selection. In pursuit of an efficient selection system, a number of separation techniques, beyond conventionally used filtration and affinity chromatography, have been tested in recent years, such as capillary electrophoresis (CE) [28], deoxyribonuclease digestion [29], surface plasmon resonance (SPR) [30], and atomic force microscopy (AFM) [31]. A particularly interesting technology is the CE-SELEX that can offer great efficiency and isolate aptamers with low dissociation constants. The first use of CE-SELEX was reported by Mendonsa and Bowser [28]. With the method, they obtained a human immunoglobulin E (IgE) aptamer by four selection rounds, with the affinity only a bit lower than that of the aptamer gained from conventional SELEX after 15 cycles. Till now, CE-SELEX has been successfully used to isolate high-affinity aptamers for large quantities of targets, such as neuropeptide Y [32] and ricin toxin [33]. Microfluidic SELEX (M-SELEX) technology may provide another valuable way to enhance the selection efficiency, considering their advantages of low cost, good reproducibility, and automation [34, 35]. By combining the magnetic bead-based separation with the microfluidic technology, Soh and coworkers have proposed a highly effective M-SELEX method [36]. In the screening process of the improved method, picomolar amounts of target protein conjugated to magnetic beads is first incubated with a random nucleic acid pool. Then, any bead-bound nucleic acids are partitioned in a disposable microfluidic chip by applying a magnetic field. After bead elution, the captured nucleic acids are amplified via PCR and used for the next cycle of selection. As a proof of principle, an aptamer against streptavidin has been generated in only three selection rounds with dissociation constants ranging from 25 to 65 nm.
Apart from purified molecules, complex targets have been increasingly adopted for the selection of aptamers. Cell-SELEX is a newly developed strategy using whole living cells as targets, which can be performed without prior knowledge of cell surface molecules [37]. As a result, a variety of aptamers capable of recognizing specific types of cells have been discovered [38–40]. Moreover, molecules on the cell membrane will keep their natural structures and contain possible posttranslational modifications throughout the Cell-SELEX process. Thus, aptamers selected using the Cell-SELEX process will be able to identify their targets with native conformations [16]. Tissues have also been used as targets for aptamer screen. Very recently, Liu et al. [41] reported a use of SELEX system to isolate aptamers specific for adipose tissue, which is considered as an important therapeutic target for obesity and related metabolic disorders.
15.3 Engineering Aptamers for Biosensor Development
A biosensor is commonly composed of at least three components, that is, a bio-recognition element, a signal-transduction system, and a physical readout instrument. Up to now, various recognition elements, including antibodies [42], receptors [43], small molecules [44], and enzymes [45], have been extensively studied and utilized for sensing. Among them, antibodies are the most commonly used ligands owing to their special high affinity and selectivity upon interacting with target molecules. However, having been developed for about 70 years, antibodies still exhibit many limitations in bioanalytical applications, such as pH and temperature sensitivity, laborious and expensive producing process, easy degradation [46]. Aptamers, which are chemically stable and cost-effective, can be promising alternatives to antibodies in the fields of biosensors. More importantly, aptamers may offer remarkable flexibility and convenience in engineering, which would further improve their properties and make them easily incorporated into novel bioassays.
15.3.1 Engineering to Improve the Bioavailability of Aptamers
Aptamers are selected in buffer systems and built from four natural DNA or RNA bases that are easily degraded by nucleases. The resulting short half-life and low binding affinity of aptamers in biological fluids would severely restrict the practical clinical application of aptamer-based biosensors (aptasensors). To overcome these problems, many efforts have been made to engineer aptamers with enhanced bioavailability [47].
Chemical modifications have been firstly introduced to different parts of aptamers to produce nuclease-resistant oligonucleotides. Inspired by nature that RNAs can be protected by methylation of the 2′-hydroxyl groups, researchers have introduced several modifications into aptamer at the 2′- position of ribose moiety, including 2′-methoxy [48], 2′-fluoro [49] and 2′-amino [50], by solid-phase-based chemical synthesis. These modifications have shown to effectively increase the stability of aptamers in complex biological fluids. Locked nucleic acids (LNAs), in which the 2′-oxygen of ribose and the 4′-carbon is bridged by a methylene group, are a novel class of modified nucleotides. Incorporation of LNAs into aptamer can provide enhanced thermal stability and good resistance against nuclease hydrolysis, without affecting the binding capability to the target. Successful applications of LNAs have been reported to a number of known aptamers, such as thrombin-binding aptamer [51] and Tenascin-C-binding aptamer [52].
From another viewpoint, the C5 position on thymine or uracil is the most permissive sites for modifications accepted by polymerases [53], laying the foundation for efficient SELEX with 5′-modified bases. In a recent study, Shoji et al. screened a thalidomide-binding aptamer from a library of non-natural DNA prepared with 5-N-(6-aminohexyl) carbamoylmethyl-2′deoxyuridine triphosphate instead of thiamine triphosphate [54]. The obtained modified DNA aptamer can be stable against several nucleases and show high enantioselectivity for the (R)-form thalidomide with a K d of 1.0 μM. In contrast to post-SELEX modifications of existing aptamers, performing SELEX on a library containing modified nucleotides may not only enhance nuclease resistance but also increase target affinity [55].
Modifications have also been introduced to the phosphodiester backbone [56]. Gorenstein and coworkers have obtained a modified DNA aptamer specific to the nuclear factor for human IL6 from a fully substituted phosphorothioate library [57]. Detailed experimental studies reveal that the modified aptamer can possess reduced nuclease activity and bind at least 5-fold tighter to the target than the normal backbone aptamer.
Another important set of modifications is performed at 3′-termini or 5′-termini of aptamers. The capping of oligonucleotide terminus has been demonstrated capable of increasing the resistance of aptamers to exonucleases. For example, an inverted thymidine cap can make the RNA aptamer against coagulation factor IXa stable in human plasma for at least 5 h [58]. Similar phenomenon may also happen to a keratinocyte growth factor aptamer modified with 2′-fluorides, 2′-Omethyl groups, and 3′-capping by inverted thymidine [59].
Other than chemical modification, multiple aptamer assembly offers an alternative way to enhance the bioavailability of aptamers through polyvalent interactions [60, 61]. Thrombin is a multifunctional serine protease with pro-coagulant and anticoagulant activities. Several aptamers have been selected against the enzyme [62–64]. One of the most intensively used aptamers is the 15-base thrombin aptamer (TBA15), which binds to the fibrinogen-binding exosite to inactivate the enzyme. Although being functional, the practical application of TBA15 is usually restricted by relatively low binding affinity. To increase the activity of TBA15, a series of methods have been proposed by engineering assemblies of TBA15 with other thrombin aptamers [65, 66]. For instance, by using a poly-dT linker, Ikebukuro and coworkers have connected the TBA15 with a 29-base aptamer (TBA29), which may recognize a different exosite of thrombin with high affinity [67]. The aptamer dimer can have a K d value of 0.15 nM, much lower than those of the individual precursor aptamers (3.5 nM for TBA29 and 20.2 nM for TBA15).
Another possible way to achieve polyvalent interactions relies on the use of nanomaterial–aptamer conjugates. One typical work conducted by Hsu et al. [68] is to covalently link the TBA15 and TBA29 to gold nanoparticles (AuNPs) through disulfide bonds together with sulfated galactose acid (sulf-Gal). Experimental results reveal that the 15-TBA15/TBA29/sulf-Gal–AuNPs conjugates can be stable in human plasma for at least 48 h and exhibit ultrahigh affinity toward thrombin (K d = 3.4 × 10−12 M). In another work, Au–Ag nanorods are utilized for multiple attachments of aptamers selected for CCRE-CEM cells [69]. Simultaneous immobilization of 80 aptamers can result in ~26-fold enhanced binding affinity compared to that of single aptamer molecule. Apart from the enhanced binding affinity, nanomaterial–aptamer conjugates can also protect the aptamer against nuclease-mediated degradation. For example, Lin and coworkers have reported an ATP biosensor that can be directly used in living cells by preparing an aptamer/graphene oxide nanocomplex [70]. Another example is a thiol-modified aptamer attached with methylene blue that is chemisorbed on AuNPs through gold–thiol bond [71]. The resulting AuNPs–aptamer conjugates can exhibit high stability during exposing to complex biofluids, proving that the immobilized aptamer is sufficiently robust for clinical diagnostic applications. Above examples clearly show that nanomaterial–aptamer conjugates are effective for fabricating aptasensors for diagnostics of biological samples [72]. With advances in nanotechnology and aptamer selection, a significant progress in this direction can be highly expected.
15.3.2 Engineering to Generate Detectable Signals
The application of aptamers in the field of biosensor mainly relies on their acting as recognition molecules with high binding affinity and specificity. However, like most other biomolecules, aptamers do not respond in any readily measurable way upon target binding [19–21]. Therefore, rational engineering of aptamers, such as incorporation with other functional nucleic acids or modification with molecular reporters, which can provide potentially solutions to the problem, may promote the development of aptasensors.
15.3.2.1 Signal Generation Incorporating Functional Nucleic Acids
Since the first report in 1996 [73], molecular beacons, defined as “nucleic acid probes able to undergo spontaneous conformational change after hybridizing their complementary strands,” have become a new kind of DNA probes that are widely used in DNA/RNA studies [74–76]. Engineering aptamers into molecular aptamer beacons (MABs) might efficiently combine the binding affinity of aptamers with the signal generation mechanism of molecular beacons. A common strategy to design MABs has been proposed by Stanton and coworkers [77], which can be used for the engineering of many aptamers. For instance, a thrombin aptamer has been engineered into a MAB by adding nucleotides to its 5′-end, complementary to a small sequence at the 3′-end of the aptamer. The hairpin structure formed in the absence of the target is then broken by the addition of thrombin, generating an increased fluorescence signal. Similar approaches have also been used to construct sensors for potassium ion [78], IgE [79], interferon-γ [80], and CCRF-CEM cells [81]. Very recently, Tan and coworkers reported a new type of MABs, named as triple helix molecular switch (THMS) [82]. The THMS consists of a dual-labeled signal-transduction probe (STP) and a central aptamer sequence that is extended with two arm segments. In the absence of target, the two arm segments of the aptamer are hybridized with the loop sequence of STP, enforcing the STP to hold an “open” configuration. However, upon target binding, the aptamer motif formes a complex with target, resulting in the release of the STP, which leads to new signal readout (Fig. 15.2). Based on this strategy, thrombin, ATP, and L-argininamide can be sensitively detected.
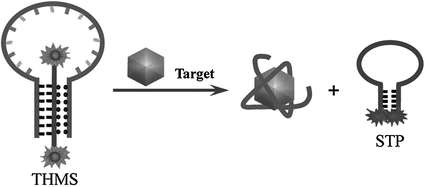
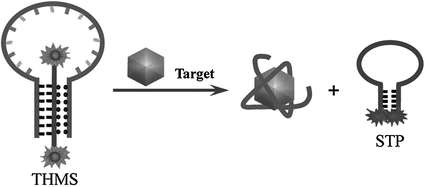
Fig. 15.2
An aptamer-based sensing platform using a triple helix molecular switch (THMS). Recreated with kind permission of American Chemical Society [82]
Catalytic nucleic acids (DNAzymes or ribozymes) are a class of nucleic acid-based biocatalysts capable of performing chemical transformations [83]. Coupling of aptamers with catalytic nucleic acids may result in novel functional nucleic acids that can be applied for both molecular recognition and signal generation in sensors. A simple way to engineer aptamers in combination with catalytic nucleic acids is to make the catalytic activity to be aptamer-target dependent [84, 85]. For instance, in a work reported by Achenbach el al. [86], an ATP aptamer is directly linked to a RNA-cleaving DNAzyme. A blocker strand is used to inhibit the catalytic activity by annealing with the aptamer–DNAzyme junction. Upon addition of ATP, however, the blocker strand could be liberated, consequently triggering the action of the DNAzyme. In a different approach, an adenosine aptamer is engineered to be embedded in the catalytic core of an Mg2+-dependent 10–23 DNAzyme [87]. The sequence of the whole DNA strand is designed to avoid forming active structure to perform cleavage of the substrate in the absence of target. However, binding of adenosine could induce a structural change of the aptamer region, activating the DNAzyme and resulting in an increased signal (Fig. 15.3).
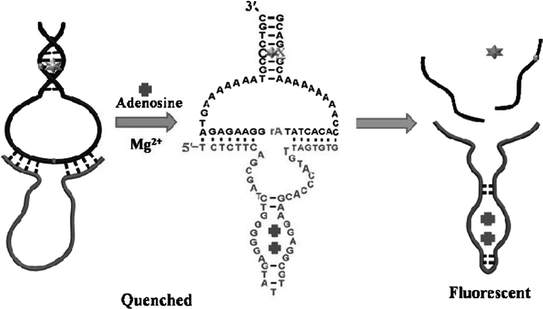
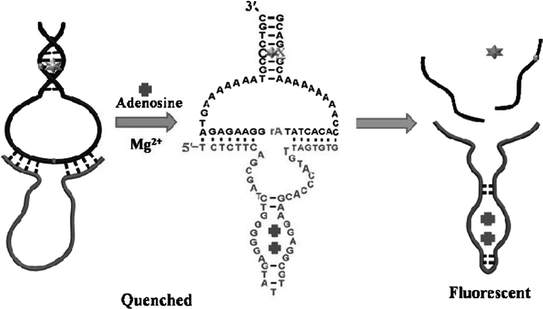
Fig. 15.3
A fluorescent aptasensor for adenosine on the basis of an aptamer–DNAzyme junction. Recreated with kind permission of American Chemical Society [87]
Besides engineering aptamers in conjugation with molecular beacons and catalytic nucleic acids, rationally designed aptamer cooperativities have also been used for biosensor development [88, 89]. Recognition and signal-transduction mechanism of such aptamer assemblies is that target binding of the recognition aptamer will induce weakening or enhancement of the binding ability of the reporting aptamer, which may subsequently generate detectable signals [24]. On the basis of positive cooperativities, Stojanovic et al. have reported a label-free fluorescent biosensor by using the aptamer against malachite green (MG) dye as a reporting element and the ATP aptamer as a recognition element [90]. Target binding by the ATP aptamer will increase the affinity and possibility for MG to bind to the reporting aptamer and exhibit high fluorescence intensity. Negative cooperativities have also been used for preparing such aptamer assemblies. For example, Wang et al. have proposed a bifunctional aptamer that contains an anti-thrombin region and a hemin–aptamer region [91]. Interestingly, the two functional regions share six crucial bases, thus making thrombin binding inhibited by hemin. In another work, the hemin–aptamer is split in two parts and fused with an adenosine aptamer and a linker sequence [92]. Binding of adenosine can force the two split of hemin–aptamer away from one another. However, once the adenosine deaminase (ADA)-catalyzed deamination process takes place, adenosine will be converted to inosine and released from the electrode, enabling the hemin–aptamer complex to be formed on the electrode surface to give an electrochemical response (Fig. 15.4). Based on this biosensor, the authors are able to assay the ADA activity with a detect limit of 0.2 U ml−1.
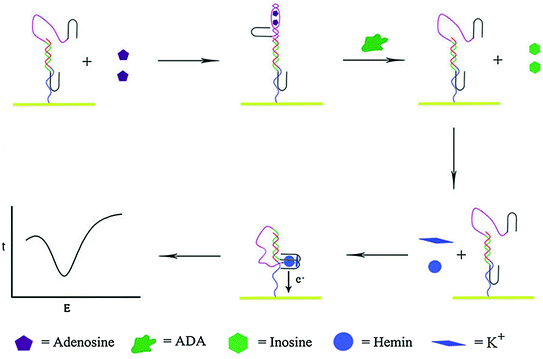
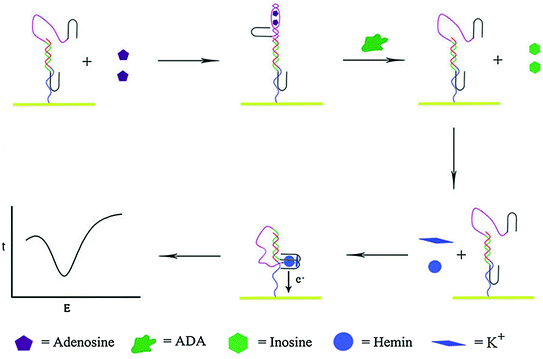
Fig. 15.4
An electrochemical sensor based on negative aptamer cooperativities. Recreated with kind permission of American Chemical Society [92]
15.3.2.2 Signal Generation Using Molecular Reporters
Through engineering aptamers with molecular reporters, the binding events can be simply converted into measureable signals. In fact, almost all sorts of reporters, including fluorophores [93], redox-active reporting units [94], proteins [95] and nanomaterials [96] can be covalently linked to aptamers. The development of reporter-linked aptamers is mainly focused on the design of different signaling strategies, so we will give an introduction on molecular reporters in the viewpoint of assay formats in this section.
In 1996, Drolet et al. detailed the first reporter-linked aptamer assay for detecting human vascular endothelial growth factor (VEGF) [97]. In this approach, a capture monoclonal antibody was immobilized on the microtiter plate surface and a VEGF aptamer was labeled on the 5′-terminus with a fluorescein moiety. In the presence of the target protein, the analyte would be captured by the antibody and the aptamer, forming a sandwich structure. Subsequently, an alkaline phosphatase-conjugated antifluorescein FAB fragment was added to bind fluorescein and generate chemiluminescence signals, which were directly concerned with the concentration of VEGF. Ever since then, sandwich designs based on reporter-linked aptamers have been widely used for biosensing, while thrombin [62, 63] and platelet-derived growth factor (PDGF) [98] that include two binding sites for the aptamers are often utilized as model targets. Figure 15.5 shows a typical work for thrombin assay. Specifically, the thrombin aptamer is covalently attached to a maleimide-functionalized siloxane monolayer. After capturing thrombin to the surface by the aptamer, AuNP-conjugated thrombin aptamer is then added to bind to the other site on thrombin [99]. The resulting AuNP seeds on the glass slides are then enlarged in the growth solution. Therefore, absorbance spectra of the functionalized surfaces can be monitored upon analyzing different concentrations of thrombin, and a detection limit of 2 nM can be achieved.
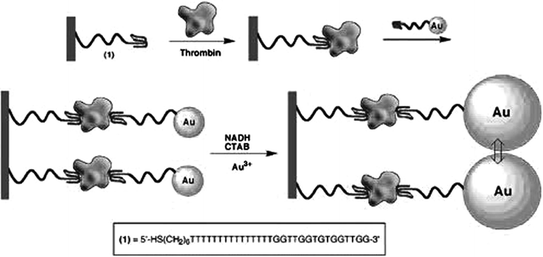
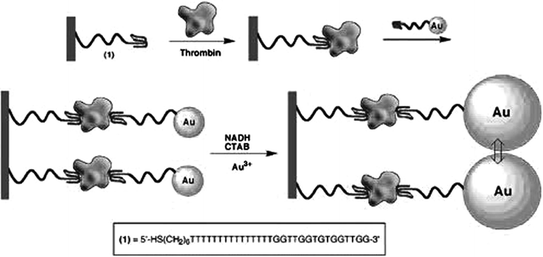
Fig. 15.5
A sandwich-type AuNP-conjugated aptamer-based colorimetric sensor for thrombin. Recreated with kind permission of American Chemical Society [99]
Sandwich structure strategy offers a feasible way to utilize reporter-linked aptamers for constructing biosensing systems with high sensitivity and specificity. However, since the assays based on sandwich structure rely on the possibility that two or more recognition molecules including aptamer can simultaneously bind to one target molecular without mutual interference, it can hardly be applied to the assays of all kinds of targets. For example, small molecules, which are usually buried within the small binding pocket of aptamer structures, usually leaving little room for the interaction with a second recognition element [100, 101], would unlikely be assayed in this format. On the other hand, proteins have complex spatial structures and they can interact with aptamers in different ways, offering a variety of possibilities for biosensor design. However, unfortunately, only a few proteins possess two recognition sites to make the recognition molecules including aptamer bind to two different epitopes [102]. So the sandwich approach based on the use of reporter-linked aptamers is mainly confined to the detection of a few kinds of proteins, such as thrombin [103, 104], PDGF [105, 106], and IgE [107].
It has been known that some aptamers will fold their flexible, single-stranded chains into unique secondary or tertiary structures when interacting with their targets. Provided the aptamers are engineered with reporter molecules, this behavior would leave the reporter moieties away from or in close proximity to quenchers for fluorescent detection, or electrodes for electrochemical detection, resulting in a detectable signal change. Therefore, the structural change of reporter-linked aptamers upon target binding has also been developed as an efficient strategy for signal generation. Fluorescence is a popular method used in this strategy. By attaching two pyrene molecules to both ends of a PDGF aptamer, Tan and coworkers have proposed a fluorescent biosensor to monitor the target protein in complex biological fluids [108]. In the absence of PDGF, the two ends of the aptamer are far away. Nevertheless, when the target exists, the target–aptamer interaction will induce aptamer conformation re-arrangement, causing the two pyrene molecules close to each other. Consequently, the fluorescence emission of the aptamer is switched from 400 to 485 nm. Such a shift in emission wavelength can solve the significant problem of background signal, allowing direct detection of PDGF in complex biological fluids.
Electrochemical means have also received increasing attentions in recent years for the use in this strategy as signal readout technique. Compared with optical assays, the sensing systems based on the fundamental of electrochemistry may be more stable, more cost-effective, easier to miniaturize, and less sensitive to contaminants [109, 110]. Therefore, emerging efforts have been made to combine the target-induced structural change of reporter-linked aptamers with different kinds of modified electrodes for biosensing. One typical example is the work conducted by Xiao et al., which is based on the fact that the thrombin aptamer will undergo a structural re-arrangement upon target binding [111]. The biosensor is constructed by covalently attaching a thrombin aptamer, dually labeled with thiol and methylene blue at either end, to a gold electrode. In the initial state, the aptamer adopts a flexible, unfolded configuration, enabling the electrical communication of methylene blue with the electrode. After interaction with thrombin, the aptamer is driven to fold into a G-quadruplex structure to make the electrochemical label away from the electrode surface, shielding the electron transfer, so the analytical signal is diminished. In a similar manner, potassium ion has also been detected with specificity [112]. In the meantime, since this sensing design has a critical disadvantage of negative signal, several “signal-on” approaches are proposed. One approach is based on the frequency tuning of the voltammetric measurements [113]. By appropriately changing the scan pulse frequency of square-wave voltammetry, the difference in the structure of target-free and target-bound aptamers can be read out electrochemically, resulting in successful “signal-on” assay of thrombin and cocaine [114]. In another approach, the interaction between the labeled aptamer and its target is rationally designed to shorten the distance of the electroactive labels from the electrode, thereby increasing the redox current. In a typical work, an anti-theophylline RNA aptamer with a terminal electroactive ferrocene (Fc) group as the redox reporter is immobilized on gold electrode (Fig. 15.6) [115]. In the absence of the analyte, the aptamer stays in a relatively open conformation, and the Fc moiety will be in various distant positions relative to the electrode surface. However, binding of theophylline will completely fold the aptamer, placing the Fc units in close proximity to the electrode surface, and thereby enhances the electron transfer efficiency, producing an increasing electrochemical current. To date, the same principle has also been applied to design sensors for the detection of thrombin [116], cocaine [117], VEGF [118], and potassium ion [119].
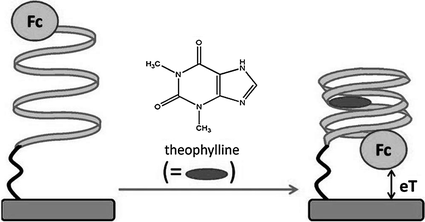
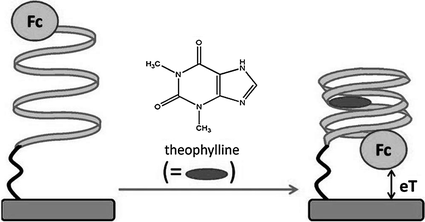
Fig. 15.6
A signal-on electrochemical aptasensor for theophylline detection by making use of the target-induced structural change of Fc-linked aptamer. Recreated with kind permission of American Chemical Society [115]
Besides the target-induced structural change strategy, rationally engineering reporter-linked aptamers into split fragments has brought a new direction for developing sensing devices. Typically, the aptamer is divided into two subunits that cannot interact with one another in the absence of the target; the specific ligand may induce self-assembly of aptamer fragments into supramolecular structures, generating a detectable signal. For the assays based on split-type strategy, small molecules are the common detection targets. Such small molecules include cocaine [120–122], ATP [123], and adenosine [124]. In addition to the small molecules, some proteins can also be assayed by using the split-type strategy [125, 126]. For instance, in a work conducted by Liu et al., an anti-thrombin aptamer is divided into two halves. While one part is immobilized on a gold electrode surface via S–Au reaction, the other is functionalized with Ru(bpy)32+-doped silica nanoparticles (Ru-SNPs) [127]. The target-directed assembly of the two fragments will make the concentration of Ru-SNPs at the electrode surface to be increased. By monitoring the electrochemiluminescence signal, thrombin can be selectively detected with a detection limit of 0.2 pM.
In the two assay strategies mentioned above, knowledge of the secondary structure of aptamers is crucial for either sensor design or split engineering. However, many aptamers cannot have an easily determined secondary structure [128]. To overcome this problem, numerous efforts have been devoted to establish alternative strategies. For instance, since aptamers can associate either with complementary DNA (cDNA) strands or with target analytes, the structure switching from an aptamer/DNA duplex to an aptamer/target complex may offer a feasible way to develop biosensors that have little restrictions on the knowledge of the secondary structure of aptamers. A representative assay conducted by Mei et al. is to use a fluorophore-linked aptamer [129]. In the resting state, fluorescence is quenched by the quencher-modified cDNA. The introduction of the target will force the departure of the cDNA strand from the aptamer, resulting in a fluorescence recovery. Using AuNPs as the quencher, Fan and coworkers have proposed a multicolor sensor, which can be used to simultaneously detect adenosine, potassium ion, and cocaine (Fig. 15.7) [130]. This strategy has also been extended to fabricate electrochemical biosensors based on the design that redox-tagged aptamer hybridizes with its complementary sequence which is immobilized on an electrode surface [131, 132]. Since the presence of the target will release the aptamer strand from the electrode, electrochemical signals are thereby changed. Of note, such electrochemical biosensors will present signal-off results, largely precluding their further development. Recently, Chen and coworkers have reported a signal-on platform for adenosine detection based on the quenching of Ru-SNPs electrochemiluminescence by ferrocene [133], which may provide a promising method to overcome the shortcoming of the signal-off methodology.
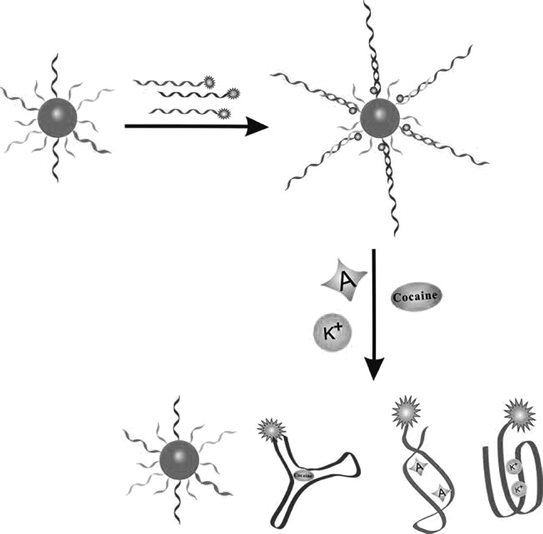
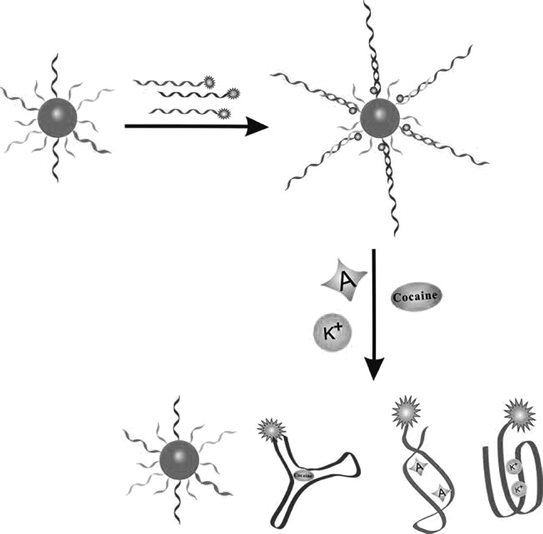
Fig. 15.7
A multicolor aptasensor based on the structure switching from an aptamer/DNA duplex to an aptamer/target complex. Recreated with kind permission of Wiley–VCH [130]
Single-stranded aptamer has been demonstrated to adsorb stably on the surface of nanomaterials, e.g., AuNPs [134], single-wall carbon nanotubes [135] and graphene oxides [136]. Interestingly, when the target is present, the aptamers will interact with their targets and be released from the nanomaterials [137–139]. On the basis of this feature, a series of aptamer sensing approaches have been generated [140–142]. Figure 15.8 shows a classical assay strategy [143]. The redox-tagged aptamers are initially bound onto the surface of magnetic graphene nanosheets (MGPs) that are adsorbed on an electrode surface. However, the interaction between the aptamer and MGPs can be easily disturbed by the formation of aptamer-target complexes. Therefore, in the presence of the targets, the redox-tagged aptamers are released from the electrode surface, resulting in decreased electrochemical signals. Through coupling DNase I-based catalytic recycling of the analytes, low detection limits (0.1 pM for ATP and 1.5 pM for cocaine) can be obtained.
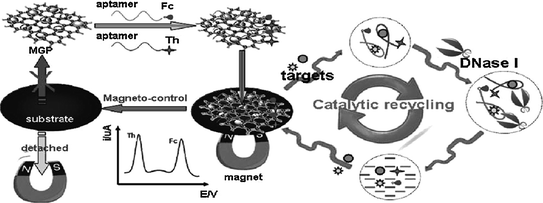
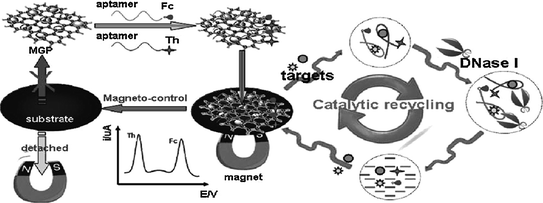
Fig. 15.8
Ultrasensitive aptamer-based multiplexed electrochemical detection with the aid of magnetic graphene nanosheets and DNase I. Recreated with kind permission of American Chemical Society [143]
15.3.3 Engineering to Achieve Signal Amplification
In some cases, the sensitivity of aptamer-based assays is not satisfactory because of the low abundance of target biomolecules in complex biofluids. For this reason, signal amplification strategies, such as nucleic acid amplification, catalytic signal amplification, and nanomaterial-mediated amplification, have been incorporated into aptasensors. Since several excellent and comprehensive reviews on signal amplification using functional nanomaterials have already been published [144, 145], only an introduction on the first two strategies is offered here.
15.3.3.1 Nucleic Acid Amplification
The chemical nature of oligonucleotides makes aptamers readily engineered to trigger nucleic acid amplification processes. As a result, a variety of sequence amplification methods, including PCR, rolling circle amplification (RCA), nicking endonuclease (NEase) or exonuclease-assisted amplification approaches, have been used in aptasensors fabrication.
PCR is one of the most powerful DNA amplification techniques and thus has been widely used in molecular diagnostic research [146, 147]. Making use of PCR can amplify a single or a few copies of a piece of DNA across several orders of magnitude, significantly improving assay sensitivity [148]. Figure 15.9 shows the utilization of PCR for IgG detection by using PCR template tethered aptamer. Firstly, the target molecules are captured on a surface, and the IgG aptamer is subjected to coupling with a double-stranded PCR template [149]. Then, direct monitoring of the PCR products may enable the fluorescent detection of the target protein with a greatly enhanced sensitivity. In another work, Landegren and coworkers have developed a novel aptamer and PCR-based strategy for protein detection, termed as proximity ligation assay (PLA) [150]. In this study, aptamers specific for PDGF are extended at both ends to be a pair of proximity probes. Upon simultaneous recognition of the same target, the extensions of such probes efficiently hybridize to a connector oligonucleotide, which is followed by the ligation of oligonucleotides and real-time PCR amplification. Given the sensitivity of the assay, PLA has also been adapted to detect other targets [151, 152].
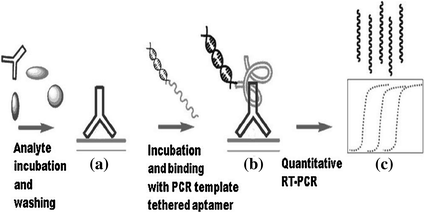
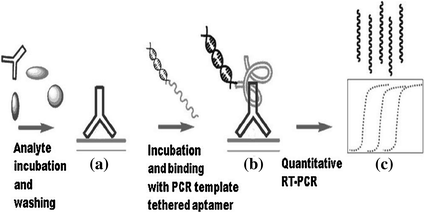
Fig. 15.9
Schematic representation of PCR-based signal amplification. Recreated with kind permission of American Chemical Society [149]
Different from PCR, RCA is an isothermal DNA amplification method that uses a short DNA primer and a circular, single-stranded template [153]. In a RCA process, DNA polymerase continuously replicates around the template and displaces the synthesized fragment, forming a long concatemeric DNA product with repeated units that are complementary to the template. Because of the needless of thermal cycling and the nuclease resistance of circular template, RCA is often employed as a signal amplification technique in clinical diagnostics [154, 155]. Adaptation of aptamers to RCA has yielded a series of sensitive sensors [156, 157]. For example, Ellington and coworkers have prepared a novel conformation-switching aptamer-based biosensor for PDGF detection [158]. Since the presence of protein target can induce circularization of the aptamer, triggering the subsequent RCA process, by monitoring the generated fluorescence signal, down to 0.4-nM PDGF can be detected.
NEases are a class of restriction endonucleases that recognize specific nucleotide sequences in double-stranded DNA but cleave only one of the strands at a fixed position [159]. The unique cleaving feature of NEase makes it favorable for performing isothermal target recycling, which enables the development of ultrasensitive detection of nucleic acids [160, 161]. In the past few years, NEase-assisted amplification has been often utilized for conducting aptamer-based assays [162, 163]. Figure 15.10a shows a typical work conducted by Zhu et al. [164]. In this system, the potassium-binding aptamer is designed to hybridize with a linker, forming two complete recognition sites for NEase. The subsequent nicking process may generate a cycle of nicking–dissociation–hybridization, resulting in the cleavage of many linkers. On the contrary, in the presence of potassium ions, the aptamer will bind with the ions, leading to the silence of NEase and the accumulation of linker molecules. By coupling the NEases-assisted amplification with probe DNA-functionalized AuNPs, potassium ion can be sensitively detected with a detection limit of ~0.1 mM. However, the above assay may hardly be extended to other analytes, due to the requirement of a NEase recognition site in the aptamer sequence. Recently, Qu and coworkers have reported a more universal strategy on the basis of a structurally engineered aptamer [165]. In this study, Qu et al. have used two hairpin structures, one of which is a MAB that contains an IgE aptamer and a trigger sequence, while another one is complementary to the trigger. Since recognition of the target will open the hairpin structure of the MAB, exposing the trigger sequence, a complete recognition site for NEase is formed, activating the autonomous nicking–dissociation–hybridization process (Fig. 15.10b). By monitoring the increase in fluorescence intensity, a detection limit of 5 pM can be achieved for IgE detection.
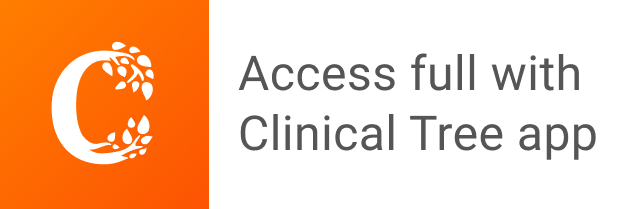