and Miguel A. R. B. Castanho2
(1)
Instituto de Bioquímica Médica Leopoldo de Meis, Federal University of Rio de Janeiro, Rio de Janeiro, Rio de Janeiro, Brazil
(2)
Institute of Biochemistry and Institute of Molecular Medicine, School of Medicine, University of Lisbon, Lisbon, Portugal
In living organisms, different types of energy are always interconverting into one another within the cell to allow the distinct cellular functions to be performed. This can be illustrated by several examples, such as the conversion of the energy of light into chemical energy in photosynthetic organisms or the chemical energy into mechanical energy in muscle contraction . Also, virtually all the cells need to use chemical energy to transport ions and other compounds across a membrane , generating a concentration gradient and thus converting chemical into osmotic energy. Finally, chemical energy is continuously converted in other forms of chemical energy during the biosynthesis of new molecules in cellular metabolism .
In the beginning of the twentieth century, a set of experiments carried out by Arthur Harden and William J. Young, in which they showed that phosphate is essential for yeast alcoholic fermentation, started a new era for the understanding on how energy is obtained from the environment and stored within the cells for later use. This discovery was the first association between phosphate and energy transformations in living cells, paving the way for the subsequent identification of ATP , more specifically its phosphoanhydride bond , as the main cellular energy carrier.
In this chapter, we will discuss the principles and the most relevant steps of the main processes of ATP synthesis in heterotrophic cells.
Heterotrophic organisms conserve the energy of nutrient molecules by coupling the breaking of their chemical bonds to the synthesis of ATP , which occurs through two distinct mechanisms.
The first mechanism of ATP synthesis to be identified is known as substrate-level phosphorylation . It does not depend on oxygen and thus may occur in anaerobiosis. The general principle involved in ATP synthesis through this mechanism is the formation of a phosphorylated molecule that presents a high-energy phosphate bond or, in a more precise term coined by Fritz Lipmann, a high potential of transferring its phosphoryl group, which is used to phosphorylate ADP , generating ATP.
The second mechanism of ATP synthesis is known as oxidative phosphorylation . It depends on organized membranes and, in eukaryotic cells, takes place in mitochondria . The unraveling of this mechanism was possible due to someone who could see beyond the energy of the chemical bonds. This man was Peter Mitchell, who postulated the revolutionary chemiosmotic theory , which states that the synthesis of ATP from ADP and inorganic phosphate (Pi ) is driven by the pH gradient across the mitochondria inner membrane and the consequent formation of a transmembrane electrical potential .
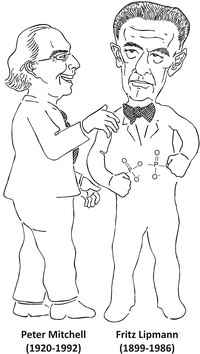
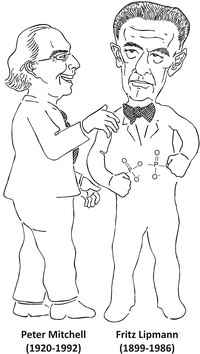
6.1 Fermentation : The Anaerobic Pathway for ATP Synthesis
As the first forms of life appeared on Earth when the atmosphere contained no oxygen yet, the anaerobic use of glucose may be considered the most ancient metabolic pathway of energy conservation.
In humans, this pathway is still very important since there are cell types that lack the oxidative apparatus, specially the mitochondria , organelles in which the oxidative phosphorylation takes place. These cells include the mature erythrocytes , cells from the crystalline, and some cells of the retina. There are also many situations in which oxygen availability is limited, and thus the ATP synthesis through fermentation becomes essential. This is the case of muscle cells in intense contraction activity, working in low oxygen conditions due to the adrenaline-induced contraction of peripheral blood vessels (see Chap. 10). There are also pathological situations in which fermentation overcomes oxidative metabolism even in the presence of oxygen. This is the case of cancer cells, in what is called the “Warburg effect ” (see Box 6.4).
It is also important to note that only sugars can be used as energy source in anaerobic conditions. The degradation of the other nutrient molecules, the lipids and the proteins , depends on metabolic pathway s that occur in mitochondria and culminate with ATP synthesis through oxidative phosphorylation , which requires oxygen as the final substrate (see details in Sect. 6.2).
6.1.1 A Historical Perspective of the Discovery of the Fermentation Process
Since ancient times, man has dealt with processes involving fermentation, although only recently fermentation has been understood as a metabolic pathway associated to the reactions of energy transformation essential for life.
Fermentation is the basis of the production of bread, beer, and wine, activities that accompanied humans since the early civilizations. The term fermentation arose from these practices, coming from the Roman word fermentare, which is related to the formation of bubbles. Thus, fermentation was firstly associated to a process in which a gas was produced. Although now we know that depending on the organism fermentation leads to different end products, which do not always include a gas, in the alcoholic fermentation, which occurs during the production of bread, beer, and wine, sugars from fruits or cereals are converted into ethanol and CO2 , which is the gas released.
Despite several advances in the scientific studies on fermentation during the nineteenth century, Louis Pasteur was the first to connect living organisms to fermentation, which was the basis for its understanding as a biological process (see Box 6.1).
Box 6.1: The Impact of Pasteur’s Ideas About Fermentation
The concept that fermentation was performed by living organisms was not readily accepted when proposed by Pasteur, especially because it seemed to reinforce the theory of vitalism, which assumed that organic substances in living organisms could be formed only under the influence of a mysterious “vital force.” Jakob Berzelius, Justus von Liebig, and Friedrich Wohler, important chemists from that time, were strongly against the vitalistic ideas, and although they were right in their concepts on the chemical processes, their convictions made them very reluctant to acknowledge the valuable contributions of Pasteur’s observations. This can be illustrated by the ironic text published by Woehler and von Liebig in 1839 in the Annals of Chemistry regarding the participation of yeast in the transformation of sugars in ethanol and CO2 during beer production: “Beer yeast, when dispersed in water, breaks down into an infinite number of small spheres. If these spheres are transferred into an aqueous solution of sugar, they develop into small animals. They are endowed with a sort of suction trunk with which they gulp the sugar from the solution. Digestion is immediate and clearly recognizable because of the discharge of excrements. These animals evacuate ethyl alcohol from their bowels and carbon dioxide from their urinary organs. Thus one can observe how a specifically lighter fluid is extruded from the anus and rises vertically, whereas a stream of carbon dioxide is ejected at very short intervals from their enormously large genitals.”
Pasteur observed microscopic globules in a wine sample (Fig. 6.1a) and sustained that these globules were living microorganisms responsible for the sugar transformation into ethanol and CO2 . He also demonstrated that each type of fermentation is linked to a specific microorganism or, as he named, a specific “ferment.” Interestingly, similar globules have been described in a sample of beer very earlier, in 1680, by Anton van Leeuwenhoek, the pioneer of the use of the microscope (Fig. 6.1b), although there is no evidence that van Leeuwenhoek had associated these globules to living organisms.
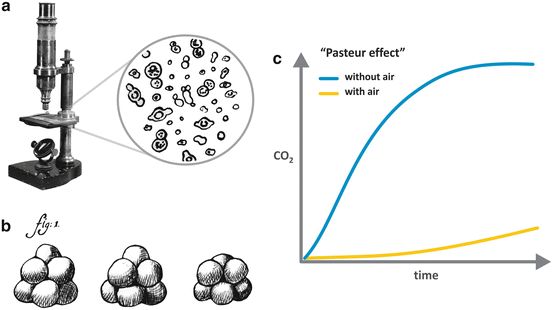
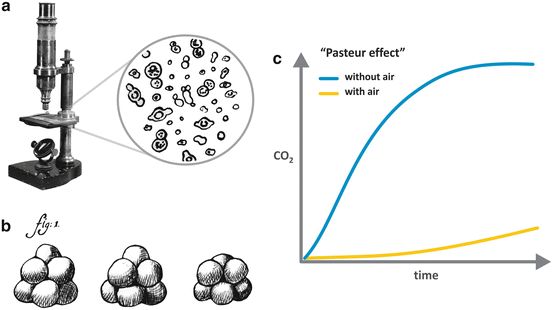
Fig. 6.1
(a) The microscope used by Pasteur to observe the wine samples and his drawing representing the “globules” he had observed in the sample (Adapted from Pasteur’s book “Études sur la bière,” 1876). (b) Drawing by van Leeuwenhoek, reporting in 1680 the observation of a beer sample in the microscope that he developed. (c) Representation of the “Pasteur effect ,” in which the production of CO2 by yeast is much higher in the absence than in the presence of oxygen
Besides associating fermentation to the presence of living organisms, Pasteur also carried out crucial experiments showing that it occurred in the absence of oxygen . These experiments discredit the current view of fermentation as a chemical process resulted from the reaction between oxygen and sugars, reinforcing the idea that fermentation was a biological process. Pasteur’s findings also allowed the formulation of other two important biological concepts derived from his experiments. The first one was that some forms of life can exist without oxygen, in what he referred in one of his most famous articles, published in 1861, as la vie sans l´air (the life without air). The second concept came from an intriguing observation that he made in those experiments: more CO2 was produced by yeast in the absence than in the presence of oxygen, a phenomenon known as the “Pasteur effect ” (Fig. 6.1c), which is now explained by the fact that the number of ATP molecules synthesized per glucose molecule in fermentation is much lower than that in oxidative phosphorylation (see in Chap. 4 the stoichiometry of ATP synthesis in the oxidative metabolism of different nutrients).
The next significant advance in the understanding of fermentation came from an unexpected observation. Eduard Buchner, working with his brother Hans in the preparation of a yeast extract to treat patients with tuberculosis, discovered that even when all the yeast cells were completely disrupted, fermentation continued to proceed normally. This finding marks the end of the vitalism theory by showing that the presence of living cells was not necessary for fermentation and can be regarded as the dawn of biochemistry, introducing the concept that biological reactions are catalyzed by molecules, which Buchner called zymases, the term firstly used to refer to what we now call enzymes . Due to the great impact of his discoveries, Eduard Buchner was the first scientist to be awarded the Nobel Prize in Chemistry, in 1907.
Finally, we must comment the experiment by Harden and Young, mentioned in the beginning of this chapter. Using the cell-free system developed by the Buchners, they observed that the fermentation rate, which decreased with time, could be restored with the addition of salts of phosphoric acid (Fig. 6.2). This was the first evidence that phosphate was involved in cell metabolism , opening the way in the subsequent years to the recognition of the ubiquitous and the crucially important biological phosphorylations in life.
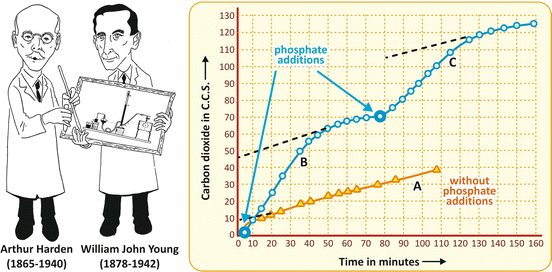
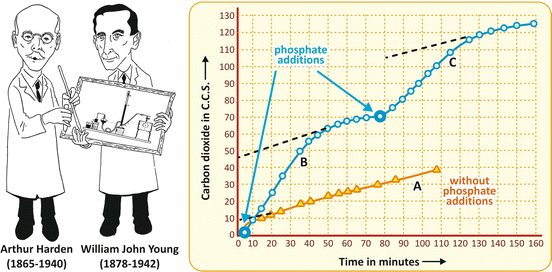
Fig. 6.2
Original figure from the article by Harden and Young published in 1906 (Adapted from Harden & Young. Proc. Royal Soc. Lond. B 77:405–520, 1906), showing the production of CO2 by yeast fermenting glucose in the absence of any addition (curve A) and when salts of phosphoric acid were added at the indicated times (curves B and C)
Few years later, Otto Meyerhof, studying muscle contraction in different organisms, showed that contraction occurred in the absence of oxygen , a similar phenomenon to that observed by Pasteur for yeast alcoholic fermentation (Fig. 6.3a). However, in the case of muscle cell fermentation, the end product was lactate. The molecular similarity of the end products of alcoholic and lactic fermentations suggested that both processes could be equivalent (Fig. 6.3b).
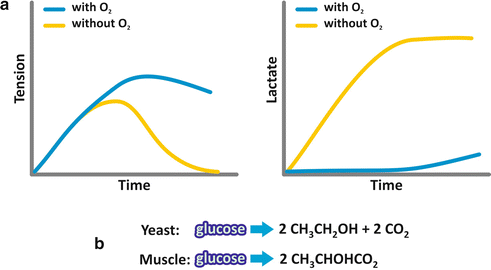
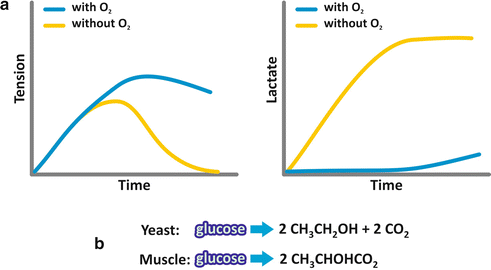
Fig. 6.3
(a) Schematic representation of the results obtained by Otto Meyerhof when a muscle fiber was incubated with glucose ; tension due to contraction and lactate production were measured as a function of time. (b) The analysis of the end products of both, alcoholic fermentation performed by yeast and lactic fermentation performed by the muscular fibers, shows great similarity
Additionally, the same requirement of phosphate was observed for fermentation in the muscle cells. Now we know that indeed the processes are identical, except for the last reaction (see next section).
6.1.2 An Overview of the ATP Synthesis by Substrate-Level Phosphorylation During Fermentation
The general mechanism of ATP synthesis that occurs during fermentation consists in a series of reactions that rearranges the molecular structure of an initially phosphorylated monosaccharide, which is a hexose phosphate, in such a way as to form phosphorylated compounds with high potential of transferring their phosphoryl group (see Box 6.2). These high-energy intermediates are triose-phosphate molecules originated from a cleavage step and whose phosphoryl group is transferred to ADP , generating ATP (Fig. 6.4a). This process of ATP synthesis is named substrate-level phosphorylation , since the phosphoryl group of an intermediate of the pathway is directly used to phosphorylate ADP.
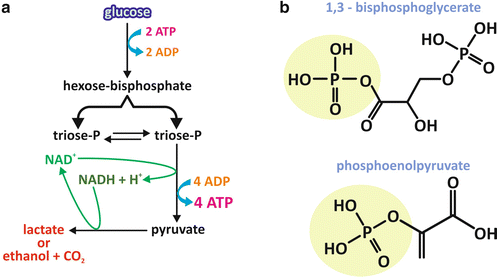
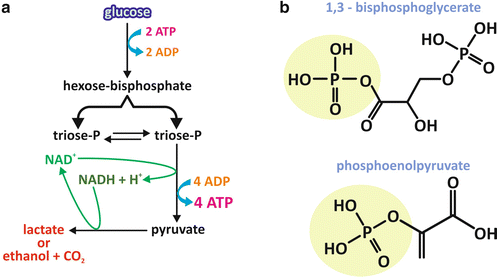
Fig. 6.4
General overview of the essentials of the fermentation process. (a) The pathway initiates with a hexose (usually glucose ). After two steps of phosphorylation with ATP as the phosphate donor, the resultant hexose bisphosphate is cleaved into two triose phosphates, which may interconvert. One of them follows the pathway, which includes one NAD+ -dependent oxidation reaction and two transfers of the phosphate group of a high-energy triose phosphate to ADP , generating ATP and, at the second of those reactions, pyruvate . Pyruvate must be reduced to allow NADH reoxidation. The main end products of fermentation are lactate or ethanol and CO2 , depending on the organism. (b) Chemical structures of the high-energy triose-phosphate molecules 1,3-bisphosphoglycerate and phosphoenolpyruvate
The initial substrate for fermentation is usually glucose , the most abundant monosaccharide derived from a regular human diet. The two initial steps of phosphorylation use ATP as the phosphoryl group donor (Fig. 6.4a). This may seem nonsensical at a first glance, if we think that this is a metabolic pathway for the synthesis of ATP. However, as it will become clear through the analysis of the stoichiometry of the entire pathway, although two ATP molecules are used for each hexose that enters the pathway in its initials steps, four ATP molecules are synthesized at the end, yielding a positive balance of two ATP molecules for each hexose molecule that is metabolized. Moreover, consumption of ATP is mandatory to “force” the first steps to occur in terms of thermodynamics (see Box 4.2).
The triose phosphates with high potential of transferring their phosphoryl group are 1,3-bisphosphoglycerate and phosphoenolpyruvate (Fig. 6.4b). In the case of 1,3-bisphosphoglycerate, an anhydride bond links the carboxyl group to the phosphate, forming what is called an acyl phosphate. In the case of phosphoenolpyruvate, the double bond between carbons 2 and 3 favors the phosphoryl group transfer.
Additionally, the pathway includes an oxidative step in which the oxidation of a three-carbon intermediate is coupled to the reduction of the coenzyme nicotinamide adenine dinucleotide (NAD+ ), generating NADH . Since the typical amount of NAD+ in the cytoplasm is much lower than the amount of glucose metabolized, fermentation should end with a step leading to the reoxidation of the NADH molecule. The reaction that does this is the one that synthesizes the end product of the pathway, which in human cells is lactate (Fig. 6.4a).
Box 6.2: The Concepts of Energy-Rich Phosphate Compounds
In his classical article from 1941, Fritz Lipmann classified the phosphate compounds as “energy rich” and “energy poor.” This classification was based on the conception that the energy released by the hydrolysis of these compounds was essentially determined by the nature of the chemical bond that links the phosphate to the molecule. Phosphoanhydride linkages generate “energy-rich” compounds, while phosphoester linkages generate “energy-poor” compounds. According to this concept, the free energy of hydrolysis of a phosphate compound would be determined essentially by the contribution of enthalpy. Due to the experimental approaches used at that time, the contribution of the environment in which the reaction takes place could not be taken into consideration, but new data obtained after 1970 introduced the idea that the energy of the hydrolysis of phosphate compounds would be also determined by the differences in their solvation energy and thus would vary greatly depending on the medium. This was found valid for compounds containing phosphoanhydride bond s , such as ATP , pyrophosphate, or acyl phosphates, but not for compounds in which the phosphate group is linked to the molecule through a phosphoester bond (see figure comparing the energy of hydrolysis of the phosphoanhydride bond of pyrophosphate with the phosphoester bond of glucose -6-phosphate as a function of water activity). Based on these observations, a new conception was formulated by the Brazilian scientist Leopoldo de Meis, according to which molecules containing phosphoanhydride bonds are susceptible to changes in the entropic energy (dependent on the solvation energy and thus on the environment), which contributes to the free energy involved in the reaction.
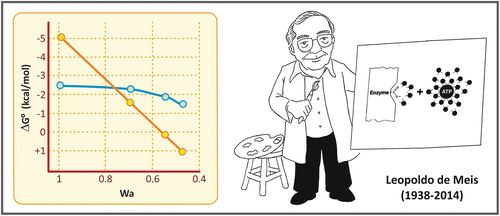
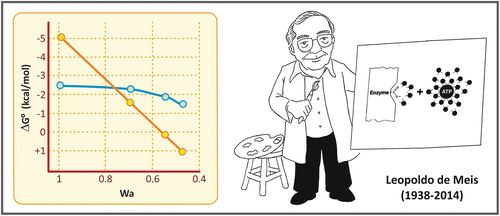
Effect of water activity (Wa) on the energy of hydrolysis of pyrophosphate (yellow circles) and glucose -6-phosphate (blue circle). Graph reproduced from de Meis in Calcium and Cellular Metabolism: Transport and Regulation, chapter 8. Plenum Press, NY, 1997
With this in mind, it is possible to understand the mechanism of ATP synthesis by ATP synthase presented in Sect. 6.2.4, in which the energy required in the reaction is not needed for the condensation of ADP and Pi , but for the ATP release from the catalytic site of the enzyme .
6.1.3 Glucose Fermentation Reactions
The first ten reactions of the fermentation process, starting with glucose and ending with the formation of two pyruvate molecules, are the same in many organisms that synthesize ATP anaerobically. The last step, which involves NADH reoxidation, is different depending on the organism, resulting in either lactate or ethanol and CO2 as the end products. Furthermore, the reactions from glucose to pyruvate are also the same that occur when carbohydrates are used aerobically (see Sect. 7.4).
The pathway from glucose to pyruvate is named glycolysis and may be divided in two parts.
In the first part, two phosphorylation steps using ATP generate a hexose with two phosphoryl groups linked, the fructose -1,6-bisphosphate , which was the compound discovered by Harden and Young (see Sect. 6.1.1), and the first intermediate of fermentation to be identified, known as the Harden–Young ester. In the first phosphorylation step, the enzyme hexokinase catalyzes the conversion of glucose in glucose-6-phosphate , which is then isomerized to fructose-6-phosphate by the phosphohexose isomerase. The second phosphorylation step is catalyzed by the phosphofructokinase and occurs at the hydroxyl group on carbon 1 of fructose-6-phosphate, generating fructose-1,6-bisphosphate. Fructose-1,6-bisphosphate is then cleaved by the aldolase into two triose-phosphate molecules, glyceraldehyde-3-phosphate and dihydroxyacetone phosphate. These triose phosphates may interconvert in a reaction catalyzed by the triose-phosphate isomerase, with dihydroxyacetone phosphate forming glyceraldehyde-3-phosphate, the next intermediate of the pathway. Thus, in the end of this first phase, one glucose molecule is converted into two glyceraldehyde-3-phosphate molecules (Fig. 6.5). In the light of the points discussed in the previous section, it is important to note that all the phosphorylated compounds of this first part of glycolysis are phosphoesters.
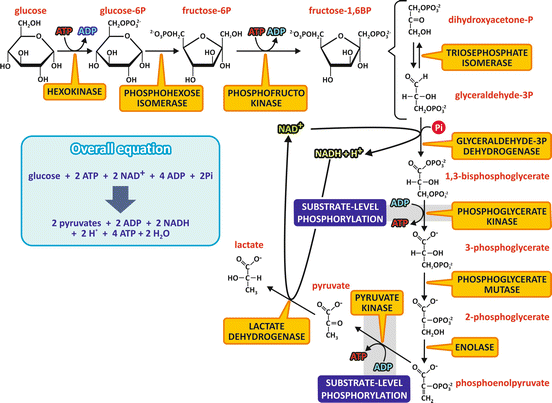
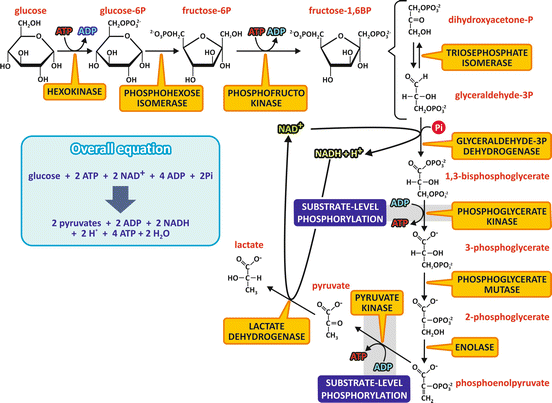
Fig. 6.5
Reactions of the fermentation of glucose to lactate. The first ten reactions consist in the metabolic pathway named glycolysis , which is also the pathway for the aerobic metabolization of carbohydrates. The last step occurs when pyruvate is not oxidized through the aerobic metabolism and NADH must be reoxidized. It should be noticed that each fructose -1,6-bisphosphate molecule leads to two glyceraldehyde -3-phosphate molecules, as dihydroxyacetone phosphate will be converted to glyceraldehyde-3-phosphate. The names of the enzymes are highlighted in yellow boxes. The steps that involve substrate-level phosphorylation are also indicated
In the second part of the pathway, each glyceraldehyde -3-phosphate molecule is oxidized in a NAD+ -dependent reaction followed by a phosphorylation step, in a reaction catalyzed by the glyceraldehyde-3-phosphate dehydrogenase , which forms 1,3-bisphosphoglycerate . Note that this phosphorylation step uses inorganic phosphate directly and not ATP as the phosphate donor. 1,3-bisphosphoglycerate is the first high-energy phosphorylated compound generated in the pathway. The oxidation of the aldehyde group of glyceraldehyde coupled to the phosphorylation reaction forms, instead of a free carboxyl group, an acyl phosphate, from which the phosphoryl group is transferred to ADP , generating ATP and 3-phosphoglycerate, in a reaction catalyzed by the phosphoglycerate kinase. Phosphoglycerate mutase converts 3-phosphoglycerate into 2-phosphoglycerate, which is then dehydrated by the enolase , forming the second compound in the pathway with high potential of transferring the phosphoryl group, the phosphoenolpyruvate (PEP ). Then, another substrate-level phosphorylation step occurs, with the phosphoryl group of PEP transferred to ADP, generating ATP and pyruvate , a reaction catalyzed by the pyruvate kinase (Fig. 6.5).
The last step in the fermentation of glucose in human cells is the reduction of pyruvate to lactate, catalyzed by the lactate dehydrogenase , allowing the reoxidation of NADH (Fig. 6.5).
When glycolysis is the means of carbohydrate utilization with concomitant use of oxygen , instead of pyruvate being reduced in the last step of fermentation, it is completely oxidized to CO2 in mitochondria (see Sect. 7.4).
6.2 Oxidative Phosphorylation: The Main Mechanism of ATP Synthesis in Most Human Cells
Oxidative phosphorylation accounts for 95 % of ATP synthesis in the human organism. This metabolic pathway is compartmentalized in mitochondria , the organelles that comprise most of the bioenergetic functions within the eukaryotic cell (see Box 6.3).
The mitochondria are unique organelles composed of two lipid membranes (Fig. 6.6). The outer mitochondrial membrane contains several porins, proteins that make membrane permeable to ions and small molecules (with molecular mass lower than 5 kDa). The inner membrane has a very high protein –lipid ratio and is rich in an unusual phospholipid , cardiolipin. It is very impermeable, with the transport of molecules and ions, including H+, occurring only through specific proteins. This membrane has a very large surface area provided by several convolutions called mitochondrial cristae (Fig. 6.6). The inner membrane encloses the mitochondrial matrix, which comprises most of the enzymes of the oxidative metabolism , the mitochondrial ribosomes, tRNA, and several copies of the mitochondrial DNA (Fig. 6.6).
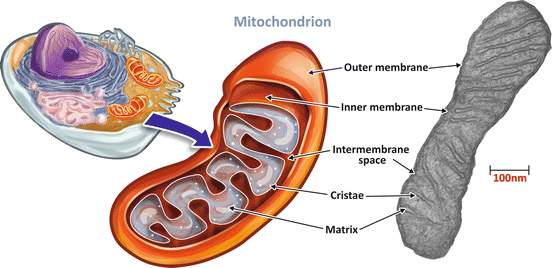
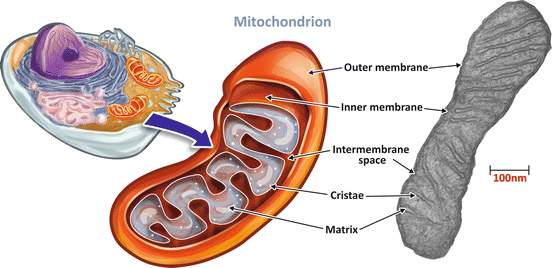
Fig. 6.6
The structure of a mitochondrion. Mitochondria are organelles formed by a permeable outer membrane and a very impermeable inner membrane with several convolution named cristae. The intramitochondrial medium forms the mitochondrial matrix. Mitochondrion transmission electron micrograph: courtesy from Prof. Marlene Benchimol
Box 6.3: The Endosymbiotic Theory for the Origin of Mitochondria
The incorporation of the mitochondria was an important event in the evolution of the eukaryotic cells. It is believed that it occurred more than 1.5 billion years ago through the invasion of a heterotrophic anaerobic cell by an aerobic bacterium. This is known as the endosymbiotic theory , which was postulated in the beginning of the twentieth century but was revived and better argued by Lynn Margulis, in the 1960s. Several genetic evidences suggest that the ancestral symbiont was an aerobic α-proteobacterium that consumed oxygen through a respiratory chain . The role of the anaerobic host in the symbiosis would be to make pyruvate accessible to the endosymbiont metabolism . On the other hand, evidence indicate that the role of the ancestral symbiont in the initial phase of the evolution of the mitochondria was to protect the anaerobic cell components from the toxic effects of oxygen through the activity of the last respiratory chain component, the enzyme cytochrome oxidase, which converts oxygen to water (see next sections of this chapter). In fact, the sharp increase in the oxygen tension around two billion years ago introduced a great threat for the anaerobic ancient cells, which did not have the detoxifying enzymes peroxidases, catalases, or superoxide dismutases that protect modern cells against the toxic effects of reactive oxygen species . Thus, the aerobic symbiont would function as an oxygen scavenger inside the host cell. With time, evolution of host genomes probably contributed with new functions to the symbiont, including the ATP /ADP transporter, transforming it into an organelle with an ATP-exporting function.
6.2.1 A Historical Perspective of the Understanding of Cellular Respiration
Since Lavoisier’s experiments in the eighteenth century, which correlated respiration to the combustion of organic matter, both processes involving oxygen consumption and CO2 release together with heat production, the concept that aerobic metabolism proceeds with the direct reaction between oxygen and the organic compounds in the body was established. However, the connection between metabolic oxidative reactions and oxygen consumption in respiration remained illusive for a long time.
In the first decades of the twentieth century, there was a serious controversy regarding the mechanisms of biological oxidations in aerobic metabolism (Fig. 6.7).
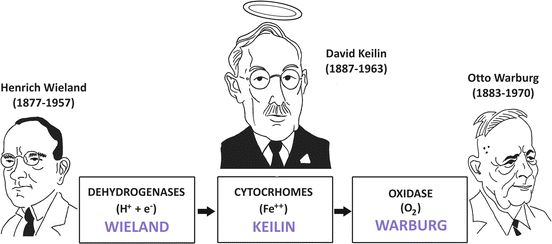
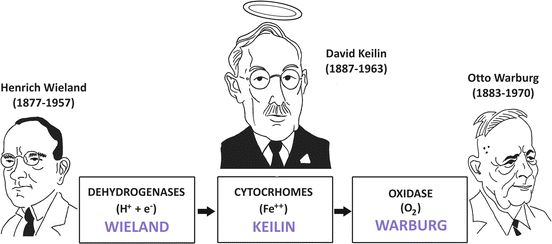
Fig. 6.7
Solution of Wieland–Warburg controversy with the discovery of the cytochromes by Keilin. Wieland postulated that the reactions catalyzed by the dehydrogenases “activated” some hydrogen atoms of the metabolic intermediates, making them labile to be transferred to a hydrogen acceptor while Warburg defended the oxygen -activating hypothesis, in which biological oxidations were catalyzed by an iron-containing enzyme . Keilin proposed that the cytochromes connected the dehydrogenases and the oxidase, being alternately reduced by the dehydrogenases and oxidized by oxygen through the Warburg enzyme
On one side, Heinrich Wieland postulated, together with Torsten Thumberg, that in the biological oxidations, the reactions catalyzed by the dehydrogenases “activated” some hydrogen atoms of the metabolic intermediates, making them labile to be transferred to a hydrogen acceptor. It is important to mention that their hypothesis, although incomplete by not taking into account the role of oxygen , correctly supported the concept that free oxygen does not directly combine with carbon to form CO2 .
On the opposite side was Otto Warburg (Box 6.4), who defended the oxygen-activating hypothesis, in which he argued that the dehydrogenase concept was unnecessary. In this hypothesis, Warburg postulated that the oxidation of all metabolites was catalyzed by an iron-containing enzyme , which he named Atmungsferment (meaning oxygen-transferring enzyme or respiratory enzyme) and in which the iron atom was oxidized to its ferric state (Fe3+) by oxygen and reduced back to its ferrous form (Fe2+) after reaction with organic substances.
The key to solve the Wieland–Warburg controversy was provided by David Keilin, who working as entomologist and parasitologist changed biochemistry with his findings (Fig. 6.7).
Box 6.4: The Diversity of Otto Warburg’s Contributions for Science
Otto Warburg was a very active and interdisciplinary scientist, giving outstanding contributions to different fields in science, including respiration , photosynthesis, and cancer cell metabolism.
Warburg strongly defended the use of quantitative methods and worked on the improvement of the instruments to get reliable measurements. Using manometric techniques, he developed an apparatus, the Warburg respirometer (see figure below), to quantify O2 uptake by thin slices of a tissue, through the changes in the chamber pressure, measured by the connection of a manometer
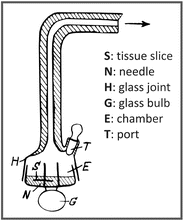
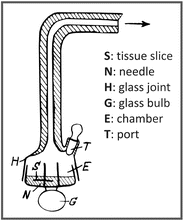
to the end of the glass joint (H). The apparatus also allowed the measurements of CO2 emission by adding, for example, potassium hydroxide in the chamber (E) to precipitate CO2. Due to his pioneering studies on cellular respiration in the beginning of the twentieth century, in which he proposed the existence of an iron-containing respiratory enzyme (the Warburg Atmungsferment), he was awarded the Nobel Prize in Physiology or Medicine in 1931.
Warburg also dedicated much time of his life investigating cancer cell metabolism . Studying different types of cancer cells in the decade of 1920, Warburg made a very intriguing observation: he found a behavior that was the opposite of the Pasteur effect (the inhibition of fermentation by O2; see Sect. 6.1.1). Warburg showed that cancer cells produced lactic acid from glucose even under aerobic conditions, which is known as the Warburg effect (see figure below). This was firstly interpreted as a consequence of a damaged respiration in cancer cells, but now we know that the Warburg effect occurs due to alterations in the regulation of glycolysis in tumorigenesis.
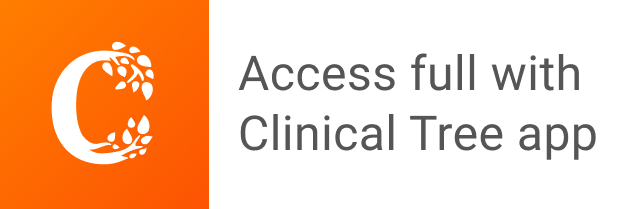