Electrolytes are ions (minerals) capable of carrying an electric charge. They are classified as either anions or cations based on the type of charge they carry. Anion and cation classifications are based on how the ion migrates in an electric field; those electrolytes with a positive charge are cations that move toward the cathode, while those with a negative charge are anions that move toward the anode.
Electrolytes are an essential component in numerous processes, including volume and osmotic regulation (sodium [Na+], chloride [Cl−], potassium [K+]); myocardial rhythm and contractility (K+, magnesium [Mg2+], calcium [Ca2+]); cofactors in enzyme activation (e.g., Mg2+, Ca2+, zinc [Zn2+]); regulation of adenosine triphosphatase (ATPase) ion pumps (Mg2+); acid–base balance (bicarbonate [HCO3–], K+, Cl−); blood coagulation (Ca2+, Mg2+); neuromuscular excitability (K+, Ca2+, Mg2+); and the production and use of ATP from glucose (e.g., Mg2+, phosphate PO4−). Because many of these functions require electrolyte concentrations to be held within very narrow ranges, the body has complex systems for monitoring and maintaining electrolyte concentrations.
This chapter explores both the metabolic physiology and regulation of each electrolyte and relates these factors to the clinical significance of electrolyte measurements. In addition, methodologies used in determining concentrations of the individual analytes are discussed.
WATER
The average water content of the human body varies from 40% to 75% of total body weight, with values declining with age and especially with obesity. Women have lower average water content than do men as a result of a higher fat content. Water is the solvent for all processes in the human body as it is responsible for transporting nutrients to cells, determining cell volume by its transport into and out of cells, removal of waste products by way of urine, and acting as the body’s coolant by way of sweating. Water is located in both intracellular and extracellular compartments. Intracellular fluid (ICF) is the fluid inside the cells and accounts for about two-thirds of total body water. Extracellular fluid (ECF) accounts for the other one-third of total body water and can be subdivided into the intravascular ECF (plasma) and the interstitial cell fluid that surrounds the cells in the tissue. Normal plasma is about 93% water, with the remaining volume occupied by lipids and proteins. The concentrations of ions within cells and in plasma are maintained both by energy-consuming active transport processes and by diffusion or passive transport processes.
Active transport is a mechanism that requires energy to move ions across cellular membranes. For example, maintaining a high intracellular concentration of K+ and a high extracellular (plasma) concentration of Na+ requires use of energy, which comes from the breakdown of ATP by ATPase-dependent ion pumps. Diffusion is the passive movement of ions (no energy consumed) across a membrane and depends on both the size and charge of the ion being transported, and on the nature of the membrane through which it is passing. The rate of diffusion of various ions also may be altered by physiologic and hormonal processes.
Distribution of water in the various body fluid compartments is controlled by maintaining the concentration of electrolytes and proteins in the individual compartments. Because most biologic membranes are freely permeable to water but not to ions or proteins, the concentration of ions and proteins on either side of the membrane will influence the flow of water across a membrane (known as an osmoregulator). In addition to the osmotic effects of Na+, other ions, proteins, and blood pressure influence the flow of water across a membrane.
Osmolality
Osmolality is a physical property of a solution that is based on the concentration of solutes (expressed as millimoles) per kilogram of solvent (w/w). Osmolality is related to several changes in the properties of a solution relative to pure water, such as freezing point depression and vapor pressure decrease. These colligative properties (see Chapter 5) are the basis for routine measurements of osmolality in the laboratory. The term osmolarity is still occasionally used, with results reported in milliosmoles per liter (w/v), but it is inaccurate in cases of hyperlipidemia or hyperproteinemia; for urine specimens; or in the presence of certain osmotically active substances, such as alcohol or mannitol. Both the sensation of thirst and arginine vasopressin hormone (AVP), formerly called antidiuretic hormone (ADH), secretion are stimulated by the hypothalamus in response to an increased osmolality of blood. The natural response to the thirst sensation is to consume more fluids, increasing the water content of the ECF, therefore diluting the elevated solute (Na+) levels and decreasing the osmolality of the plasma. Thirst, therefore, is important in mediating fluid intake. The other means of controlling osmolality is by secretion of AVP. This hormone is secreted by the posterior pituitary gland and acts on the cells of the collecting ducts in the kidneys to increase water reabsorption. This is an example of a negative feedback loop; as water is conserved, the osmolality decreases, which in turn shuts off AVP secretion (feedback loops are discussed more in Chapter 20).1
Clinical Significance of Osmolality
Osmolality in plasma is important because it is the parameter to which the hypothalamus responds. The regulation of osmolality also affects the Na+ concentration in plasma, largely because Na+ and its associated anions account for approximately 90% of the osmotic activity in plasma. Another important process affecting the Na+ concentration in blood is the regulation of blood volume. As discussed later, although osmolality and volume are regulated by separate mechanisms (except for AVP and thirst), they are related because osmolality (Na+) is regulated by changes in water balance, whereas volume is regulated by changes in Na+ balance.1
To maintain a normal plasma osmolality (275 to 295 mOsm/kg of plasma H2O), osmoreceptors in the hypothalamus respond quickly to small changes in osmolality. A 1% to 2% increase in osmolality causes a fourfold increase in the circulating concentration of AVP, and a 1% to 2% decrease in osmolality shuts off AVP production. AVP acts by increasing the reabsorption of water in the cortical and medullary collecting tubules but has a half-life in the circulation of only 15 to 20 minutes.
Renal water regulation by AVP and thirst play important roles in regulating plasma osmolality. Renal water excretion is more important in controlling water excess, whereas thirst is more important in preventing water deficit (dehydration). Consider what happens in several conditions.
Water Load
As excess intake of water (e.g., in polydipsia) begins to lower plasma osmolality, both AVP and thirst are suppressed. In the absence of AVP, water is not reabsorbed causing a large volume of dilute urine to be excreted; as much as 10 to 20 L of water daily can be excreted, well above any normal intake of water. Therefore, hypoosmolality and hyponatremia usually occur only in patients with impaired renal excretion of water.1
Water Deficit
As the deficit of water begins to increase plasma osmolality, both AVP secretion and thirst are activated. Although AVP contributes by minimizing renal water loss, thirst is the major defense against hyperosmolality and hypernatremia. Although hypernatremia rarely occurs in a person with a normal thirst mechanism and access to water, it becomes a concern in infants, unconscious patients, or anyone who is unable to either drink or ask for water. Osmotic stimulation of thirst progressively diminishes in people who are older than age 60. In the older patient with illness and diminished mental status, dehydration becomes increasingly likely. An example of the effectiveness of thirst in preventing dehydration can be seen in patients with diabetes insipidus, where there is either no AVP production or no ability to respond to the AVP in circulation. In these patients, excretion may increase to 10 L of urine per day; however, because thirst persists, water intake matches output and plasma Na+, and therefore plasma osmolality, remain normal.1
Regulation of Blood Volume
Adequate blood volume is essential to maintain blood pressure and ensure good perfusion to all tissues and organs. Regulation of both Na+ and water is interrelated in controlling blood volume. The renin–angiotensin–aldosterone hormone system responds primarily to a decreased blood volume. Renin is secreted near the renal glomeruli in response to decreased renal blood flow (decreased blood volume or blood pressure). Renin converts the hormone angiotensinogen to angiotensin I, which then becomes angiotensin II. Angiotensin II causes vasoconstriction, which quickly increases blood pressure, and secretion of aldosterone, which increases retention of Na+ and the water that accompanies the Na+. The effects of blood volume and osmolality on Na+ and water metabolism are shown in Figure 16.1. Changes in blood volume (which is actually a change in pressure) are initially detected by a series of stretch receptors located in areas such as the cardiopulmonary circulation, carotid sinus, aortic arch, and glomerular arterioles. These receptors then activate a series of responses (effectors) that restore volume by appropriately varying vascular resistance, cardiac output, and renal Na+ and water retention.1
FIGURE 16.1 Responses to changes in blood osmolality and blood volume. ANP, atrial natriuretic peptide; ADH, antidiuretic hormone; ACE, angiotensin-converting enzyme. The primary stimuli are shown in boxes (e.g., hypovolemia).
Four other factors affect blood volume: (1) atrial natriuretic peptide (ANP), released from the myocardial atria in response to volume expansion, promotes Na+ excretion in the kidney (B-type natriuretic peptide and ANP act together in regulating blood pressure and fluid balance); (2) volume receptors independent of osmolality stimulate the release of AVP, which conserves water by renal reabsorption; (3) glomerular filtration rate (GFR) increases with volume expansion and decreases with volume depletion; and (4) all other things equal, an increased plasma Na+ will increase urinary Na+ (and therefore water) excretion and vice versa. The normal reabsorption of 98% to 99% of filtered Na+ by the tubules conserves nearly all of the 150 L of glomerular filtrate produced daily, while a 1% to 2% reduction in tubular reabsorption of Na+ can increase water loss by several liters per day.
Urine osmolality values may vary widely depending on water intake and the circumstances of collection. However, it is generally decreased in diabetes insipidus and polydipsia (excessive H2O intake) and increased in conditions such as the syndrome of inappropriate antidiuretic hormone (SIADH) secretion and hypovolemia (although urinary Na+ is usually decreased).
Determination of Osmolality
Specimen
Osmolality may be measured in serum or urine. Major electrolyte concentrations, mainly sodium, chloride, and bicarbonate, provide the largest contribution to the osmolality value of serum. Plasma use is not recommended because osmotically active substances may be introduced into the specimen from the anticoagulant.
Discussion
The methods for determining osmolality are based on properties of a solution that are related to the number of molecules of solute per kilogram of solvent (colligative properties), such as changes in freezing point and vapor pressure. An increase in osmolality decreases both the freezing point temperature and the vapor pressure. Measurements of freezing point depression and vapor pressure decrease (actually, the dew point) are the two most frequently used methods of analysis. For detailed information on theory and methodology, consult Chapter 5 or the operator’s manual of the instrument being used.
Samples must be free of particulate matter to obtain accurate results. Turbid serum and urine samples should be centrifuged before analysis to remove any extraneous particles. If reusable sample cups are used, they should be thoroughly cleaned, disinfected, and dried between each use to prevent contamination.
Osmometers that operate by freezing point depression are standardized using sodium chloride reference solutions. After calibration, the appropriate amount of sample is pipetted into the required cuvette or sample cup and placed in the analyzer. The sample is then supercooled to −7°C and seeded to initiate the freezing process. When temperature equilibrium has been reached, the freezing point is measured, with results for serum and urine osmolality reported as milliosmoles per kilogram.
Calculation of osmolality has some usefulness either as an estimate of the true osmolality or to determine the osmolal gap, which is the difference between the measured osmolality and the calculated osmolality. The osmolal gap indirectly indicates the presence of osmotically active substances other than Na+, urea, or glucose, such as ethanol, methanol, ethylene glycol, isopropanol, lactate, or β-hydroxybutyrate.
Two formulas are presented, each having theoretic advantages and disadvantages. Both are adequate for the purpose previously described. For more discussion, the reader may consult other references.2
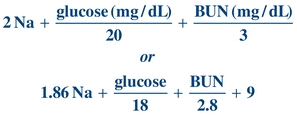
Reference Ranges
See Table 16.1.3
TABLE 16.1 Reference Ranges for Osmolality
THE ELECTROLYTES
Sodium
Na+ is the most abundant cation in the ECF, representing 90% of all extracellular cations, and largely determines the osmolality of the plasma. A normal plasma osmolality is approximately 295 mmol/L, with Na+ and associated anions accounting for 270 mmol/L.
Na+ concentration in the ECF is much larger than inside the cells. Because a small amount of Na+ can diffuse through the cell membrane, the two sides would eventually reach equilibrium. To prevent equilibrium and to ensure a concentration gradient, active transport systems, such as ATPase ion pumps, are present in all cells. K+ (see section “Potassium”) is the major intracellular cation. Like Na+, K+ would eventually diffuse across the cell membrane until equilibrium is reached. The Na+/K+-ATPase ion pump moves three Na+ ions out of the cell in exchange for two K+ ions moving into the cell as ATP is converted to ADP. Because water follows electrolytes across cell membranes, the continual removal of Na+ from the cell prevents osmotic rupture of the cell by also drawing water from the cell.
Regulation
The plasma Na+ concentration depends greatly on the intake and excretion of water and, to a somewhat lesser degree, on the renal regulation of Na+. Three processes are of primary importance: (1) the intake of water in response to thirst, as stimulated or suppressed by plasma osmolality; (2) the excretion of water, largely affected by AVP release in response to changes in either blood volume or osmolality; and (3) the blood volume status, which affects Na+ excretion through aldosterone, angiotensin II, and ANP. The kidneys have the ability to conserve or excrete large amounts of Na+, depending on the Na+ content of the ECF and the blood volume. Normally, 60% to 75% of filtered Na+ is reabsorbed in the proximal tubule; electroneutrality is maintained by either Cl− reabsorption or hydrogen ion (H+) secretion. Some Na+ is also reabsorbed in the loop and distal tubule and (controlled by aldosterone) exchanged for K+ in the connecting segment and cortical collecting tubule. The regulation of osmolality and volume has been summarized in Figure 16.1.
CASE STUDY 16.1
A 32-year-old woman was admitted to the hospital following 2½ days of severe vomiting. Before this episode, she was reportedly well. Physical findings revealed decreased skin turgor and dry mucous membranes. Admission study results were as follows:
Serum
- Na+: 129 mmol/L
- K+: 5.0 mmol/L
- Cl−: 77 mmol/L
- HCO3−: 9 mmol/L
- Osmolality: 265 mOsm/kg
Urine
- Na+: 8 mmol/d
- Ketones: trace
Questions
1. What is the cause for each abnormal plasma electrolyte result?
2. What is the significance of the urine sodium and serum osmolality results?
Clinical Applications
Hyponatremia
Hyponatremia is defined as a serum/plasma level less than 135 mmol/L, and levels below 130 mmol/L are clinically significant.4 Hyponatremia is one of the most common electrolyte disorders in hospitalized and nonhospitalized patients and can be assessed either by the cause of the decrease or with the osmolality level.5,6
Decreased levels may be caused by increased Na+ loss, increased water retention, or water imbalance (Table 16.2). Increased Na+ loss in the urine can occur with decreased aldosterone production, certain diuretics (thiazides), ketonuria (Na+ lost with ketones), or a salt-losing nephropathy (with some renal tubular disorders). K+ deficiency also causes Na+ loss because of the inverse relationship of the two ions in the renal tubules; when serum K+ levels are low, the renal tubules will conserve K+ and excrete Na+ in exchange for the loss of the monovalent cation. Each disorder results in an increased urine Na+ level (≥20 mmol/d), which exceeds the amount of water loss.7
TABLE 16.2 Causes of Hyponatremia
SIADH, syndrome of inappropriate arginine vasopressin hormone secretion.
Prolonged vomiting, diarrhea, or severe burns can result in Na+ loss. Urine Na+ levels are usually less than 20 mmol/d in these disorders, which can be used to differentiate among causes for urinary loss.
Increased water retention causes dilution of serum/plasma Na+ as with acute or chronic renal failure. In nephrotic syndrome and hepatic cirrhosis, plasma proteins are decreased, resulting in a decreased colloid osmotic pressure in which intravascular fluid migrates to the tissue (edema results). The low plasma volume causes AVP to be produced, causing fluid retention and resulting in dilution of Na+. This compensatory mechanism is also seen with congestive heart failure (CHF) as a result of increased venous pressure. Urine Na+ levels can be used to differentiate the cause for increased water retention; when urine Na+ is ≥20 mmol/d, acute or chronic renal failure is the likely cause, and when urine levels are less than 20 mmol/d, water retention may be a result of nephrotic syndrome, hepatic cirrhosis, or CHF.7
Water imbalance can occur as a result of excess water intake, as with polydipsia (increased thirst). The increased intake must be chronic before water imbalance occurs, which may cause mild or severe hyponatremia. In a normal individual, excess intake will not affect Na+ levels. SIADH causes an increase in water retention because of increased AVP (ADH) production. A defect in AVP regulation has been associated with pulmonary disease, malignancies, central nervous system (CNS) disorders, infections (e.g., Pneumocystis carinii pneumonia), or trauma.7 Pseudohyponatremia can occur when Na+ is measured using indirect ion-selective electrodes (ISEs) in a patient who is hyperproteinemic or hyperlipidemic. An indirect ISE dilutes the sample prior to analysis, and as a result of serum/plasma water displacement, the ion levels are falsely decreased. (For detailed information on the theory of water displacement with indirect ISEs, consult Chapter 5.)
Hyponatremia can also be classified according to serum/plasma osmolality (Table 16.3). Because Na+ is a major contributor to osmolality, both levels can assist in identifying the cause of hyponatremia. There are three categories of hyponatremia—low osmolality, normal osmolality, or high osmolality.4 Most instances of hyponatremia occur with decreased osmolality. This may be a result of Na+ loss or water retention, as previously mentioned.
TABLE 16.3 Classification of Hyponatremia by Osmolality
Hyponatremia with a normal osmolality may be a result of a high increase in nonsodium cations as listed in Table 16.4. In multiple myeloma, the cationic γ-globulins replace some Na+ to maintain the electroneutrality; however, because it is a multivalent cation, it has little effect on osmolality.
TABLE 16.4 Causes of Hypernatremia
Pseudohyponatremia, as mentioned earlier, may also be seen with in vitro hemolysis, considered the most common cause for a false decrease.4 When red blood cells (RBCs) lyse, Na+, K+, and water are released, and since Na+ concentration is lower in RBCs, this results in a false decrease. Hyponatremia with a high osmolality is associated with hyperglycemia. The elevated levels of glucose increase the serum osmolality and cause a shift of water from the cells to the blood, resulting in a dilution of Na+.
Symptoms of hyponatremia
Symptoms depend on the serum level. Between 125 and 130 mmol/L, symptoms are primarily gastrointestinal (GI). More severe neuropsychiatric symptoms are seen below 125 mmol/L, including nausea and vomiting, muscular weakness, headache, lethargy, and ataxia. More severe symptoms also include seizures, coma, and respiratory depression.7 A level below 120 mmol/L for 48 hours or less (acute hyponatremia) is considered a medical emergency.8 Serum and urine electrolytes are monitored as treatment to return Na+ levels to normal occurs.9
Treatment of hyponatremia
Treatment is directed at correction of the condition that caused either water loss or Na+ loss in excess of water loss. In addition, the onset of hyponatremia—acute or chronic (less than or more than 48 hours)—and the severity of hyponatremia are considered in treatment. Conventional treatment of hyponatremia involves fluid restriction and providing hypertonic saline and/or other pharmacologic agents that may take several days to reach the desired effect and may have deleterious side effects.5 Correcting severe hyponatremia too rapidly can cause cerebral myelinolysis and too slowly can cause cerebral edema.9 Appropriate management of fluid administration is critical. Fluid administration and monitoring are required during treatment of the underlying cause of the hyponatremia.
A newer type of pharmacologic agent, an AVP receptor antagonist, has been found to be an effective treatment for euvolemic or hypervolemic hyponatremia. Conivaptan has been approved by the U.S. Food and Drug Administration for use in the United States and blocks the action of AVP in the collecting ducts of the nephron, thus decreasing water reabsorption.10 This AVP receptor antagonist tends to restore Na+ levels within 24 hours.5 Euvolemic hypernatremia is associated with SIADH, hypothyroidism, and adrenal insuffiency.6 Hypervolemic hyponatremia is associated with liver cirrhosis with ascites, CHF, and overhydrated postoperative patients.8 Conivaptan is not an effective treatment with hypovolemic hyponatremia because the increased water loss would accentuate the volume depletion problem.10
Hypernatremia
Hypernatremia (increased serum Na+ concentration) results from excess loss of water relative to Na+ loss, decreased water intake, or increased Na+ intake or retention. Hypernatremia is less commonly seen in hospitalized patients than is hyponatremia.7
Loss of hypotonic fluid may occur either by the kidney or through profuse sweating, diarrhea, or severe burns.
Hypernatremia may result from loss of water in diabetes insipidus, either because the kidney cannot respond to AVP (nephrogenic diabetes insipidus) or because AVP secretion is impaired (central diabetes insipidus). Diabetes insipidus is characterized by copious production of dilute urine (3 to 20 L/d). Because people with diabetes insipidus drink large volumes of water, hypernatremia usually does not occur unless the thirst mechanism is also impaired. Partial defects of either AVP release or the response to AVP may also occur. In such cases, urine is concentrated to a lesser extent than appropriate to correct the hypernatremia. Excess water loss may also occur in renal tubular disease, such as acute tubular necrosis, in which the tubules become unable to fully concentrate the urine.
The measurement of urine osmolality is necessary to evaluate the cause of hypernatremia. With renal loss of water, the urine osmolality is low or normal. With extrarenal fluid losses, the urine osmolality is increased. Interpretation of the urine osmolality in hypernatremia is shown in Table 16.5.
TABLE 16.5 Hypernatremia (150 mmol/L) Related to Urine Osmolality
AVP, arginine vasopressin hormone.
Water loss through the skin and by breathing (insensible loss) accounts for about 1 L of water loss per day in adults. Any condition that increases water loss, such as fever, burns, diarrhea, or exposure to heat, will increase the likelihood of developing hypernatremia. Commonly, hypernatremia occurs in those persons who may be thirsty but who are unable to ask for or obtain water, such as adults with altered mental status and infants. When urine cannot be fully concentrated (e.g., in neonates, young children, older persons, and certain patients with renal insufficiency), a relatively lower urine osmolality may occur.
Chronic hypernatremia in an alert patient is indicative of hypothalamic disease, usually with a defect in the osmoreceptors rather than from a true resetting of the osmostat. A reset osmostat may occur in primary hyperaldosteronism, in which excess aldosterone induces mild hypervolemia that retards AVP release, shifting plasma Na+ upward by approximately 3 to 5 mmol/L.1
Hypernatremia may be from excess ingestion of salt or administration of hypertonic solutions of Na+, such as sodium bicarbonate or hypertonic dialysis solutions. Neonates are especially susceptible to hypernatremia from this cause. In these cases, AVP response is appropriate, resulting in urine osmolality of greater than 800 mOsm/kg (Table 16.5).
Symptoms of hypernatremia
Symptoms most commonly involve the CNS as a result of the hyperosmolar state. These symptoms include altered mental status, lethargy, irritability, restlessness, seizures, muscle twitching, hyperreflexes, fever, nausea or vomiting, difficult respiration, and increased thirst. Serum Na+ of more than 160 mmol/L is associated with a mortality rate of 60% to 75%.7
Treatment of hypernatremia
Treatment is directed at correction of the underlying condition that caused the water depletion or Na+ retention. The speed of correction depends on the rate with which the condition developed. Hypernatremia must be corrected gradually because too rapid a correction of serious hypernatremia (≥160 mmol/L) can induce cerebral edema and death; the maximal rate should be 0.5 mmol/L/h.1
Determination of Sodium
Specimen
Serum, plasma, and urine are all acceptable for Na+ measurements. When plasma is used, lithium heparin, ammonium heparin, and lithium oxalate are suitable anticoagulants. Hemolysis does not cause a significant change in serum or plasma values as a result of decreased levels of intracellular Na+. However, with marked hemolysis, levels may be decreased as a result of a dilutional effect.
Whole blood samples may be used with some analyzers; however, consult the instrument operation manual for acceptability. The specimen of choice in urine Na+ analyses is a 24-hour collection. Sweat is also suitable for analysis, and its collection and analysis are discussed in Chapter 29.
Methods
Through the years, Na+ has been measured in various ways, including chemical methods, flame emission spectrophotometry, atomic absorption spectrophotometry (AAS), and ISEs. Chemical methods are outdated because of large sample volume requirements and lack of precision. ISEs are the most routinely used method in clinical laboratories.
The ISE method uses a semipermeable membrane to develop a potential produced by having different ion concentrations on either side of the membrane. In this type of system, two electrodes are used. One electrode has a constant potential, making it the reference electrode, and the difference in potential between the reference and measuring electrodes can be used to calculate the “concentration” of the ion in solution. However, it is the activity of the ion, not the concentration that is being measured (see Chapter 5). Most analyzers use a glass ion-exchange membrane in its ISE system for Na+ measurement (Fig. 16.2). There are two types of ISE measurement, based on sample preparation: direct and indirect. Direct measurement provides an undiluted sample to interact with the ISE membrane. With the indirect method, a diluted sample is used for measurement. There is no significant difference in results, except when samples are hyperlipidemic or hyperproteinemic. In these samples, excess lipids or proteins displace plasma water, which leads to a falsely decreased measurement of ionic activity in millimoles per liter of plasma, whereas the direct method measures in plasma water only. In these cases, direct ISE is more accurate.
FIGURE 16.2 Diagram of sodium ISE with glass capillary membrane. (Courtesy of Nova Biomedical, Waltham, MA.)
One source of error with ISEs is protein buildup on the membrane through continuous use. The protein-coated membranes cause poor selectivity, which results in poor reproducibility of results. A routine maintenance of these ISEs requires removal of this protein buildup to ensure quality results.
VITROS analyzers (Ortho-Clinical Diagnostics) use a single-use direct ISE potentiometric system. Each disposable slide contains a reference and measuring electrode (Fig. 16.3). A drop of sample fluid and a drop of reference fluid are simultaneously applied to the slide, and the potential difference between the two is measured, which is proportional to the Na+ concentration.11
FIGURE 16.3 Schematic diagram of the ISE system for the potentiometric slide on the VITROS. (Courtesy of OCD, a Johnson & Johnson company, Rochester, NY.)
Reference Ranges
See Table 16.6.3
TABLE 16.6 Reference Ranges for Sodium
Potassium
Potassium (K+) is the major intracellular cation in the body, with a concentration 20 times greater inside the cells than outside. Many cellular functions require that the body maintain a low ECF concentration of K+ ions. As a result, only 2% of the body’s total K+ circulates in the plasma. Functions of K+ in the body include regulation of neuromuscular excitability, contraction of the heart, ICF volume, and H+ concentration.1
The K+ concentration has a major effect on the contraction of skeletal and cardiac muscles. An elevated plasma K+ decreases the resting membrane potential (RMP) of the cell (the RMP is closer to zero), which decreases the net difference between the cell’s resting potential and threshold (action) potential. A lower than normal difference increases cell excitability, leading to muscle weakness. Severe hyperkalemia can ultimately cause a lack of muscle excitability (as a result of a higher RMP than action potential), which may lead to paralysis or a fatal cardiac arrhythmia.1 Hypokalemia decreases cell excitability by increasing the RMP, often resulting in an arrhythmia or paralysis.1 The heart may cease to contract in extreme cases of either hyperkalemia or hypokalemia.
K+ concentration also affects the H+ concentration in the blood. For example, in hypokalemia (low serum K+), as K+ is lost from the body, Na+ and H+ move into the cell. The H+ concentration is, therefore, decreased in the ECF, resulting in alkalosis.
Regulation
Renal function related to tubular reabsorption and secretion is important in the regulation of potassium balance. Initially, the proximal tubules reabsorb nearly all the K+. Then, under the influence of aldosterone, additional K+ is secreted into the urine in exchange for Na+ in both the distal tubules and the collecting ducts. Thus, the distal nephron is the principal determinant of urinary K+ excretion. Most individuals consume far more K+ than needed; the excess is excreted in the urine but may accumulate to toxic levels if renal failure occurs.
K+ uptake from the ECF into the cells is important in normalizing an acute rise in plasma K+ concentration due to an increased K+ intake. Excess plasma K+ rapidly enters the cells to normalize plasma K+. As the cellular K+ gradually returns to the plasma, it is removed by urinary excretion. Note that chronic loss of cellular K+ may result in cellular depletion before there is an appreciable change in the plasma K+ concentration because excess K+ is normally excreted in the urine.
Three factors that influence the distribution of K+ between cells and ECF are as follows: (1) K+ loss frequently occurs whenever the Na+, K+-ATPase pump is inhibited by conditions such as hypoxia, hypomagnesemia, or digoxin overdose; (2) insulin promotes acute entry of K+ into skeletal muscle and liver by increasing Na+, K+-ATPase activity; and (3) catecholamines, such as epinephrine (β2-stimulator), promote cellular entry of K+, whereas propanolol (β-blocker) impairs cellular entry of K+. Dietary deficiency or excess is rarely a primary cause of hypokalemia or hyperkalemia. However, with a preexisting condition, dietary deficiency (or excess) can enhance the degree of hypokalemia (or hyperkalemia).
Exercise
K+ is released from muscle cells during exercise, which may increase plasma K+ by 0.3 to 1.2 mmol/L with mild to moderate exercise and by as much as 2 to 3 mmol/L with exhaustive exercise. These changes are usually reversed after several minutes of rest. Forearm exercise during venipuncture can cause erroneously high plasma K+ concentrations.12
Hyperosmolality
Hyperosmolality, as with uncontrolled diabetes mellitus, causes water to diffuse from the cells, carrying K+ with the water, which leads to gradual depletion of K+ if kidney function is normal.
Cellular Breakdown
Cellular breakdown releases K+ into the ECF. Examples are severe trauma, tumor lysis syndrome, and massive blood transfusions.
Clinical Applications
Hypokalemia
Hypokalemia is a plasma K+ concentration below the lower limit of the reference range. Hypokalemia can occur with GI or urinary loss of K+ or with increased cellular uptake of K+. Common causes of hypokalemia are shown in Table 16.7. Of these, therapy with thiazide-type diuretics is the most common.13 GI loss occurs when GI fluid is lost through vomiting, diarrhea, gastric suction, or discharge from an intestinal fistula. Increased K+ loss in the stool also occurs with certain tumors, malabsorption, cancer therapy (chemotherapy or radiation therapy), and large doses of laxatives.
TABLE 16.7 Causes of Hypokalemia
Renal loss of K+ can result from kidney disorders such as K+-losing nephritis and renal tubular acidosis (RTA). In RTA, as tubular excretion of H+ decreases, K+ excretion increases. Because aldosterone promotes Na+ retention and K+ loss, hyperaldosteronism can lead to hypokalemia and metabolic alkalosis.1 Hypomagnesemia can lead to hypokalemia by promoting urinary loss of K+. Mg2+ deficiency also diminishes the activity of Na+, K+-ATPase and enhances the secretion of aldosterone. Effective treatment requires supplementation with both Mg2+ and K+.1 Renal K+ loss also occurs with acute myelogenous leukemia, acute myelomonocytic leukemia, and acute lymphocytic leukemia.13 Although reduced dietary intake of K+ rarely causes hypokalemia in healthy persons, decreased intake may intensify hypokalemia caused by use of diuretics, for example.
Increased cellular uptake of potassium is encountered in alkalemia and with elevated levels of insulin via therapeutic treatment of diabetes. Both alkalemia and insulin increase the cellular uptake of K+. Because alkalemia promotes intracellular loss of H+ to minimize elevation of intracellular pH, both K+ and Na+ enter cells to preserve electroneutrality. Plasma K+ decreases by about 0.4 mmol/L per 0.1 unit rise in pH.1
Insulin promotes the entry of K+ into skeletal muscle and liver cells. Because insulin therapy can sometimes uncover an underlying hypokalemic state, plasma K+ should be monitored carefully whenever insulin is administered to susceptible patients.1 A rare cause of hypokalemia is associated with a blood sample from a leukemic patient with a significantly elevated white blood cell count as the K+ present in the sample is taken up by the white cells if the sample is left at room temperature for several hours.13
Symptoms of hypokalemia
Symptoms (e.g., weakness, fatigue, and constipation) often become apparent as plasma K+ decreases below 3 mmol/L. Hypokalemia can lead to muscle weakness or paralysis, which can interfere with breathing. The dangers of hypokalemia concern all patients, but especially those with cardiovascular disorders because of an increased risk of arrhythmia, which may cause sudden death in certain patients. Mild hypokalemia (3.0 to 3.4 mmol/L) is usually asymptomatic.
Treatment of hypokalemia
Treatment typically includes oral KCl replacement of K+ over several days. In some instances, intravenous (IV) replacement may be indicated. In some cases, chronic mild hypokalemia may be corrected simply by including food in the diet with high K+ content, such as dried fruits, nuts, bran cereals, bananas, and orange juice. Plasma electrolytes are monitored as treatment to return K+ levels to normal occurs.
Hyperkalemia
The most common causes of hyperkalemia are shown in Table 16.8. Patients with hyperkalemia often have an underlying disorder, such as renal insufficiency, diabetes mellitus, or metabolic acidosis, that contributes to hyperkalemia.12 For example, during administration of KCl, a person with renal insufficiency is far more likely to develop hyperkalemia than is a person with normal renal function. The most common cause of hyperkalemia in hospitalized patients is due to therapeutic K+ administration. The risk is greatest with IV K+ replacement.12
TABLE 16.8 Causes of Hyperkalemia
GFR, glomerular filtration rate.
In healthy persons, an acute oral load of K+ will briefly increase plasma K+ because most of the absorbed K+ rapidly moves intracellularly. Normal cellular processes gradually release this excess K+ back into the plasma, where it is normally removed by renal excretion. Impairment of urinary K+ excretion is usually associated with chronic hyperkalemia.1
If a shift of K+ from cells into plasma occurs too rapidly to be removed by renal excretion, acute hyperkalemia develops. In diabetes mellitus, insulin deficiency promotes cellular loss of K+. Hyperglycemia also contributes by producing a hyperosmolar plasma that pulls water and K+ from cells, promoting further loss of K+ into the plasma.1 In metabolic acidosis, as excess H+ moves intracellularly to be buffered, K+ leaves the cell to maintain electroneutrality. Plasma K+ increases by 0.2 to 1.7 mmol/L for each 0.1 unit reduction of pH.1 Because cellular K+ often becomes depleted in cases of acidosis with hyperkalemia (including diabetic ketoacidosis), treatment with agents such as insulin and bicarbonate can cause a rapid intracellular movement of K+, producing severe hypokalemia.
Various drugs may cause hyperkalemia, especially in patients with either renal insufficiency or diabetes mellitus. These drugs include captopril (inhibits angiotensin-converting enzyme), nonsteroidal anti-inflammatory agents (inhibit aldosterone), spironolactone (K+-sparing diuretic), digoxin (inhibits Na+–K+ pump), cyclosporine (inhibits renal response to aldosterone), and heparin therapy (inhibits aldosterone secretion).
Hyperkalemia may result when K+ is released into the ECF during enhanced tissue breakdown or catabolism, especially if renal insufficiency is present. Increased cellular breakdown may be caused by trauma, administration of cytotoxic agents, massive hemolysis, tumor lysis syndrome, and blood transfusions. In banked blood, K+ is gradually released from erythrocytes during storage, often causing elevated K+ concentration in plasma supernatant.
Patients on cardiac bypass may develop mild elevations in plasma K+ during warming after surgery because warming causes cellular release of K+, whereas hypothermia causes movement of K+ into cells.
Symptoms of hyperkalemia
Hyperkalemia can cause muscle weakness, tingling, numbness, or mental confusion by altering neuromuscular conduction. Muscle weakness does not usually develop until plasma K+ reaches 8 mmol/L.1
Hyperkalemia disturbs cardiac conduction, which can lead to cardiac arrhythmias and possible cardiac arrest. Plasma K+ concentrations of 6 to 7 mmol/L may alter the electrocardiogram (ECG), and concentrations more than 10 mmol/L may cause fatal cardiac arrest.1
Treatment of hyperkalemia
Treatment should be immediately initiated when serum K+ is 6.0 to 6.5 mmol/L or greater or if there are ECG changes.12 To offset the effect of K+, which lowers the resting potential of myocardial cells, Ca2+ may be given to reduce the threshold potential of myocardial cells. Therefore, Ca2+ provides immediate but short-lived protection to the myocardium against the effects of hyperkalemia. Substances that acutely shift K+ back into cells, such as sodium bicarbonate, glucose, or insulin, may also be administered. K+ may be quickly removed from the body by use of diuretics (loop), if renal function is adequate, or sodium polystyrene sulfonate (Kayexalate) enemas, which bind to K+ secreted in the colon. Hemodialysis can be used if other measures fail.12 Patients treated with these agents must be monitored carefully to prevent hypokalemia as K+ moves back into cells or is removed from the body.
Collection of Samples
Proper collection and handling of samples for K+ analysis is extremely important because there are many causes of artifactual hyperkalemia. First, the coagulation process releases K+ from platelets, so that serum K+ may be 0.1 to 0.7 mmol/L higher than plasma K+ concentrations.2 If the patient’s platelet count is elevated (thrombocytosis), serum K+ may be further elevated. Second, if a tourniquet is left on the arm too long during blood collection or if patients excessively clench their fists or otherwise exercise their forearms before venipuncture, cells may release K+ into the plasma. The first situation may be avoided by using a heparinized tube to prevent clotting of the specimen and the second by using proper care in the drawing of blood. Third, because storing blood on ice promotes the release of K+ from cells, whole blood samples for K+ determinations should be stored at room temperature (never iced) and analyzed promptly or centrifuged to remove the cells.14 Fourth, if hemolysis occurs after the blood is drawn, K+ may be falsely elevated—the most common cause of artifactual hyperkalemia. Slight hemolysis (≈50 mg/dL of hemoglobin) can cause an increase of approximately 3%, while gross hemolysis (>500 mg/dL of hemoglobin) can cause an increase of up to 30%.2
Determination of Potassium
Specimen
Serum, plasma, and urine may be acceptable for analysis. Hemolysis must be avoided because of the high K+ content of erythrocytes. Heparin is the anticoagulant of choice. Whereas serum and plasma generally give similar K+ levels, serum reference intervals tend to be slightly higher. Significantly elevated platelet counts may result in the release of K+ during clotting from rupture of these cells, causing a spurious hyperkalemia. In this case, plasma is preferred. Whole blood samples may be used with some analyzers; however, one should consult the instrument’s operations manual for acceptability. Urine specimens should be collected over a 24-hour period to eliminate the influence of diurnal variation.
Methods
As with Na+, the current method of choice is ISE. For ISE measurements, a valinomycin membrane is used to selectively bind K+, causing an impedance change that can be correlated to K+ concentration where KCl is the inner electrolyte solution.
Reference Ranges
See Table 16.9.3
TABLE 16.9 Reference Ranges for Potassium
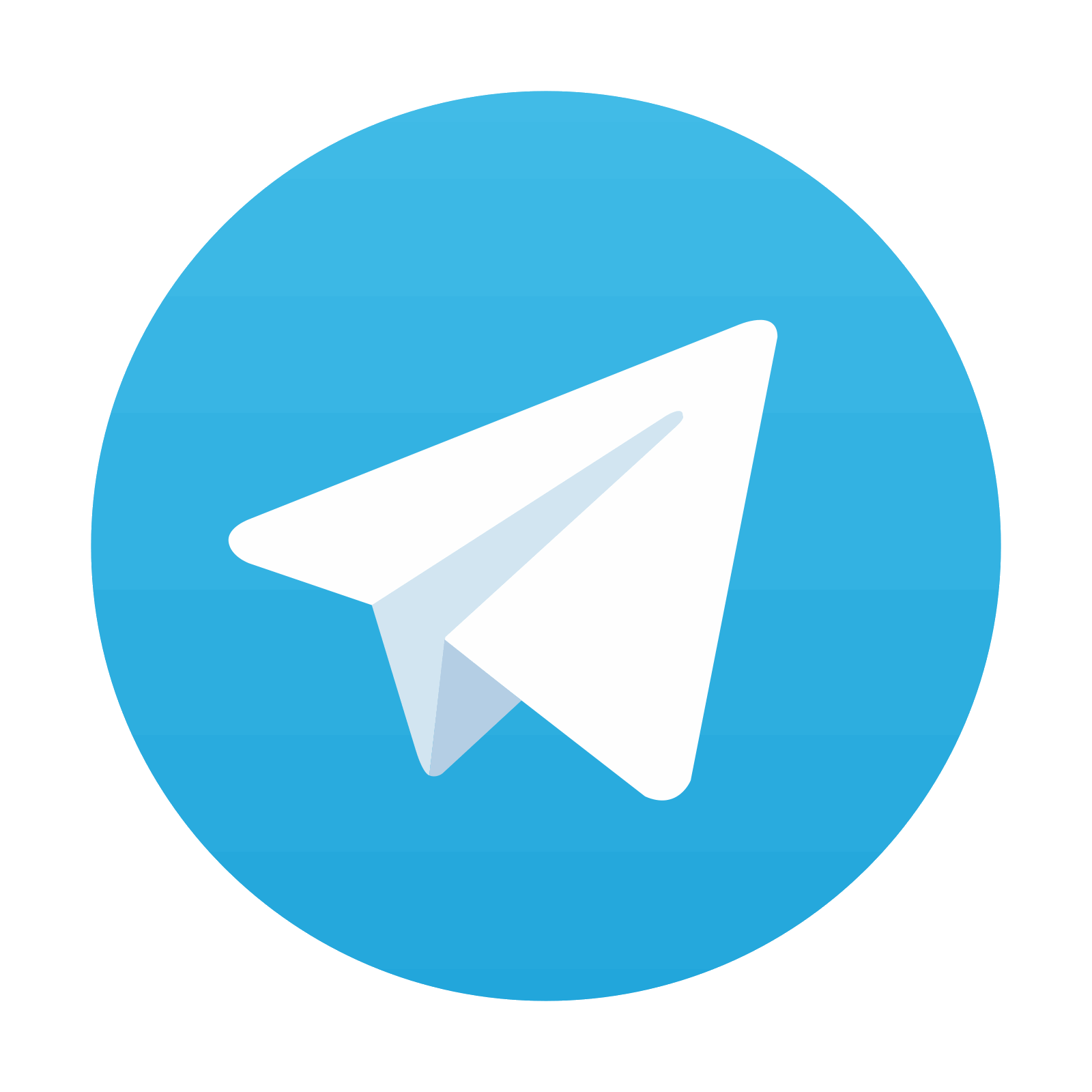
Stay updated, free articles. Join our Telegram channel
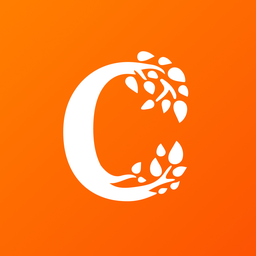
Full access? Get Clinical Tree
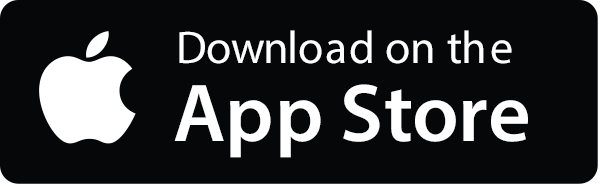
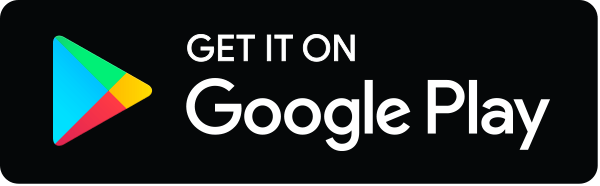