Drug Invention and the Pharmaceutical Industry
The first edition of Goodman & Gilman helped to organize the field of pharmacology, giving it intellectual validity and an academic identity. That edition began: “The subject of pharmacology is a broad one and embraces the knowledge of the source, physical and chemical properties, compounding, physiological actions, absorption, fate, and excretion, and therapeutic uses of drugs. A drug may be broadly defined as any chemical agent that affects living protoplasm, and few substances would escape inclusion by this definition.” This General Principles section provides the underpinnings for these definitions by exploring the processes of drug invention, followed by the basic properties of the interactions between the drug and biological systems: pharmacodynamics, pharmacokinetics (including drug transport and metabolism), and pharmacogenomics. Subsequent sections deal with the use of drugs as therapeutic agents in human subjects.
Use of the term invention to describe the process by which a new drug is identified and brought to medical practice, rather than the more conventional term discovery, is intentional. The term invention emphasizes the process by which drugs are sculpted and brought into being based on experimentation and optimization of many independent properties; there is little serendipity.
FROM EARLY EXPERIENCES WITH PLANTS TO MODERN CHEMISTRY
The human fascination—and sometimes infatuation—with chemicals that alter biological function is ancient and results from long experience with and dependence on plants. Many plants produce harmful compounds for defense that animals have learned to avoid and humans to exploit.
Examples are described in earlier editions of this text: the appreciation of coffee (caffeine) by the prior of an Arabian convent who noted the behavior of goats that gamboled and frisked through the night after eating the berries of the coffee plant; the use of mushrooms and the deadly nightshade plant by professional poisoners; of belladonna (“beautiful lady”) to dilate pupils; of the Chinese herb ma huang (containing ephedrine) as a circulatory stimulant; of curare by South American Indians to paralyze and kill animals hunted for food; and of poppy juice (opium) containing morphine (from the Greek Morpheus, the god of dreams) for pain relief and control of dysentery. Morphine, of course, has well-known addicting properties, mimicked in some ways by other problematic (“recreational”) natural products—nicotine, cocaine, and ethanol.
Although terrestrial and marine organisms remain valuable sources of compounds with pharmacological activities, drug invention became more allied with synthetic organic chemistry as that discipline flourished over the past 150 years, beginning in the dye industry. Dyes are colored compounds with selective affinity for biological tissues. Study of these interactions stimulated Paul Ehrlich to postulate the existence of chemical receptors in tissues that interacted with and “fixed” the dyes. Similarly, Ehrlich thought that unique receptors on microorganisms or parasites might react specifically with certain dyes and that such selectivity could spare normal tissue. Ehrlich’s work culminated in the invention of arsphenamine in 1907, which was patented as “salvarsan,” suggestive of the hope that the chemical would be the salvation of humankind. This and other organic arsenicals were invaluable for the chemotherapy of syphilis until the discovery of penicillin. Through the work of Gerhard Domagk, another dye, prontosil (the first clinically useful sulfonamide), was shown to be dramatically effective in treating streptococcal infections, launching the era of antimicrobial chemotherapy. The collaboration of pharmacology with chemistry on the one hand, and with clinical medicine on the other, has been a major contributor to the effective treatment of disease, especially since the middle of the 20th century.
SOURCES OF DRUGS
SMALL MOLECULES ARE THE TRADITION
With the exception of a few naturally occurring hormones (e.g., insulin), most drugs were small organic molecules (typically <500 Da) until recombinant DNA technology permitted synthesis of proteins by various organisms (bacteria, yeast) and mammalian cells. The usual approach to invention of a small-molecule drug is to screen a collection of chemicals (“library”) for compounds with the desired features. An alternative is to synthesize and focus on close chemical relatives of a substance known to participate in a biological reaction of interest (e.g., congeners of a specific enzyme substrate chosen to be possible inhibitors of the enzymatic reaction), a particularly important strategy in the discovery of anticancer drugs.
Drug discovery in the past often resulted from serendipitous observations of the effects of plant extracts or individual chemicals on animals or humans; today’s approach relies on high-throughput screening of libraries containing hundreds of thousands or even millions of compounds for their capacity to interact with a specific molecular target or elicit a specific biological response. Ideally, the target molecules are of human origin, obtained by transcription and translation of the cloned human gene. The potential drugs that are identified in the screen (“hits”) are thus known to react with the human protein and not just with its relative (ortholog) obtained from mouse or another species.
Among the variables considered in screening are the “drugability” of the target and the stringency of the screen in terms of the concentrations of compounds that are tested. “Drugability” refers to the ease with which the function of a target can be altered in the desired fashion by a small organic molecule. If the protein target has a well-defined binding site for a small molecule (e.g., a catalytic or allosteric site), chances are excellent that hits will be obtained. If the goal is to employ a small molecule to mimic or disrupt the interaction between 2 proteins, the challenge is much greater.
FROM HITS TO LEADS
Initial hits in a screen are rarely marketable drugs, often having modest affinity for the target, and lacking the desired specificity and pharmacological properties. Medicinal chemists synthesize derivatives of the hits, thereby defining the structure–activity relationship and optimizing parameters such as affinity for the target, agonist/antagonist activity, permeability across cell membranes, absorption and distribution in the body, metabolism, and unwanted effects.
This approach was driven largely by instinct and trial and error in the past; modern drug development frequently takes advantage of determination of a high-resolution structure of the putative drug bound to its target. X-ray crystallography offers the most detailed structural information if the target protein can be crystallized with the lead drug bound to it. Using techniques of molecular modeling and computational chemistry, the structure provides the chemist with information about substitutions likely to improve the “fit” of the drug with the target and thus enhance the affinity of the drug for its target. Nuclear magnetic resonance (NMR) studies of the drug-receptor complex also can provide useful information, with the advantage that the complex need not be crystallized.
The holy grail of this approach to drug invention is to achieve success entirely through computation. Imagine a database containing detailed chemical information about millions of chemicals and a second database containing detailed structural information about all human proteins. The computational approach is to “roll” all the chemicals over the protein of interest to find those with high-affinity interactions. The dream gets bolder if we acquire the ability to roll the chemicals that bind to the target of interest over all other human proteins to discard compounds that have unwanted interactions. Finally, we also will want to predict the structural and functional consequences of a drug binding to its target (a huge challenge), as well as all relevant pharmacokinetic properties of the molecules of interest. Indeed, computational approaches have suggested new uses for old drugs and offered explanations for recent failures of drugs in the later stages of clinical development (e.g., torcetrapib; see below).
LARGE MOLECULES ARE INCREASINGLY IMPORTANT
Protein therapeutics were uncommon before the advent of recombinant DNA technology. Insulin was introduced into clinical medicine for the treatment of diabetes following the experiments of Banting and Best in 1921. Insulins purified from porcine or bovine pancreas are active in humans, although antibodies to the foreign proteins are occasionally problematic. Growth hormone, used to treat pituitary dwarfism, exhibits more stringent species specificity: only the human hormone could be used after purification from pituitary glands harvested during autopsy, and such use had its dangers. Some patients who received the human hormone developed Creutzfeldt-Jakob disease (the human equivalent of mad cow disease), a fatal degenerative neurological disease caused by prion proteins that contaminated the drug preparation. Thanks to gene cloning and the production of large quantities of proteins by expressing the cloned gene in bacteria or eukaryotic cells, protein therapeutics now use highly purified preparations of human (or humanized) proteins. Rare proteins can be produced in quantity, and immunological reactions are minimized. Proteins can be designed, customized, and optimized using genetic engineering techniques. Other types of macromolecules may also be used therapeutically. For example, antisense oligonucleotides are used to block gene transcription or translation, as are small interfering RNAs (siRNAs).
Proteins used therapeutically include hormones, growth factors (e.g., erythropoietin, granulocyte-colony stimulating factor), cytokines, and a number of monoclonal antibodies used in the treatment of cancer and autoimmune diseases. Murine monoclonal antibodies can be “humanized” (by substituting human for mouse amino acid sequences). Alternatively, mice have been engineered by replacement of critical mouse genes with their human equivalents, such that they make completely human antibodies. Protein therapeutics are administered parenterally, and their receptors or targets must be accessible extracellularly.
TARGETS OF DRUG ACTION
Early drugs came from observation of the effects of plants after their ingestion by animals, with no knowledge of the drug’s mechanism and site of action. Although this approach is still useful (e.g., in screening for the capacity of natural products to kill microorganisms or malignant cells), modern drug invention usually takes the opposite approach—starting with a statement (or hypothesis) that a certain protein or pathway plays a critical role in the pathogenesis of a certain disease, and that altering the protein’s activity would therefore be effective against that disease. Crucial questions arise:
• Can one find a drug that will have the desired effect against its target?
• Does modulation of the target protein affect the course of disease?
• Does this project make sense economically?
The effort expended to find the desired drug will be determined by the degree of confidence in the answers to the latter 2 questions.
IS THE TARGET DRUGABLE?
The drugability of a target with a low-molecular-weight organic molecule relies on the presence of a binding site for the drug that exhibits considerable affinity and selectivity.
If the target is an enzyme or a receptor for a small ligand, one is encouraged. If the target is related to another protein that is known to have, for example, a binding site for a regulatory ligand, one is hopeful. However, if the known ligands are large peptides or proteins with an extensive set of contacts with their receptor, the challenge is much greater. If the goal is to disrupt interactions between 2 proteins, it may be necessary to find a “hot spot” that is crucial for the protein-protein interaction, and such a region may not be detected. Accessibility of the drug to its target also is critical. Extracellular targets are intrinsically easier to approach and, in general, only extracellular targets are accessible to macromolecular drugs.
HAS THE TARGET BEEN VALIDATED?
This question is obviously a critical one. A negative answer, frequently obtained only retrospectively, is a common cause of failure in drug invention.
Biological systems frequently contain redundant elements, or can alter expression of drug-regulated elements to compensate for the effect of the drug. In general, the more important the function, the greater the complexity of the system. For example, many mechanisms control feeding and appetite, and drugs to control obesity have been notoriously difficult to find. The discovery of the hormone leptin, which suppresses appetite, was based on mutations in mice that cause loss of either leptin or its receptor; either kind of mutation results in enormous obesity in both mice and people. Leptin thus appeared to be a marvelous opportunity to treat obesity. However, obese individuals have high circulating concentrations of leptin and appear quite insensitive to its action.
Modern techniques of molecular biology offer powerful tools for validation of potential drug targets, to the extent that the biology of model systems resembles human biology. Genes can be inserted, disrupted, and altered in mice. One can thereby create models of disease in animals or mimic the effects of long-term disruption or activation of a given biological process. If, for example, disruption of the gene encoding a specific enzyme or receptor has a beneficial effect in a valid murine model of a human disease, one may believe that the potential drug target has been validated. Mutations in humans also can provide extraordinarily valuable information. For example, loss-of-function mutations in the PCSK9 gene (encoding proprotein convertase subtilisin/kexin type 9) greatly lower concentrations of LDL cholesterol in plasma and reduce the risk of myocardial infarction. Based on these findings, many drug companies are actively seeking inhibitors of PCSK9 function.
IS THIS DRUG INVENTION EFFORT ECONOMICALLY VIABLE?
Drug invention and development is expensive (see below), and economic realities influence the direction of pharmaceutical research.
For example, investor-owned companies generally cannot afford to develop products for rare diseases or for diseases that are common only in economically underdeveloped parts of the world. Funds to invent drugs targeting rare diseases or diseases primarily affecting developing countries (especially parasitic diseases) usually come from taxpayers or very wealthy philanthropists.
ADDITIONAL PRECLINICAL RESEARCH
Following the path just described can yield a potential drug molecule that interacts with a validated target and alters its function in the desired fashion. Now one must consider all aspects of the molecule in question—its affinity and selectivity for interaction with the target, its pharmacokinetic properties (absorption, distribution, metabolism, excretion: ADME), issues of its large-scale synthesis or purification, its pharmaceutical properties (stability, solubility, questions of formulation), and its safety. One hopes to correct, to the extent possible, any obvious deficiencies by modification of the molecule itself or by changes in the way the molecule is presented for use.
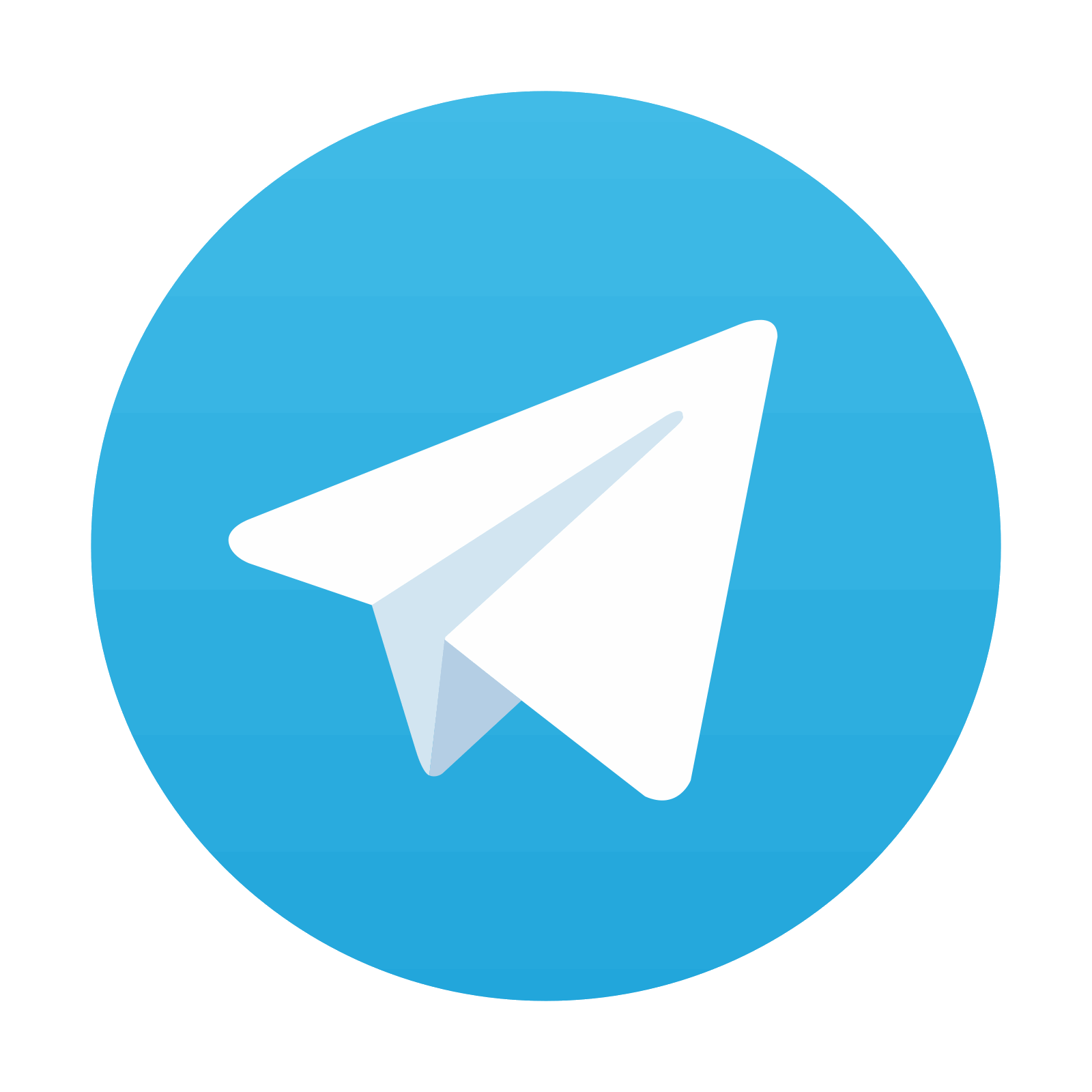
Stay updated, free articles. Join our Telegram channel
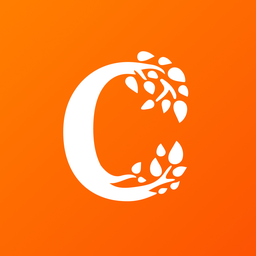
Full access? Get Clinical Tree
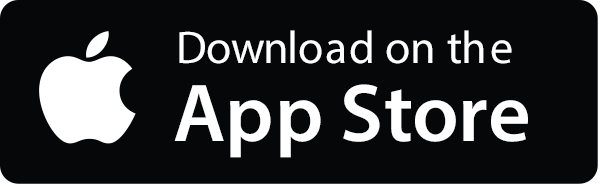
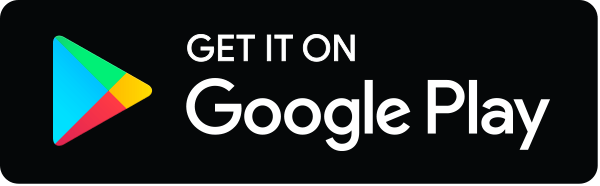