Armelle Leturque, PhD and Edith Brot-Laroche, PhD∗ Carbohydrates typically provide 45% to 65% of energy in human diets. Dietary carbohydrates include a variety of simple and complex carbohydrates, some of which are digestible by human gastrointestinal enzymes and some of which are not hydrolyzed by these enzymes. The digestible carbohydrates give rise to monosaccharide units that are absorbed by sugar transport systems. The processes involved in the digestion and absorption of digestible carbohydrates are the focus of this chapter. Dietary fiber is not a source of glucose or other monosaccharides in the small intestine. Dietary fiber is made up mainly of complex nondigestible carbohydrates and resistant starches from plant foods. The fate of the nondigestible carbohydrates is discussed in detail in Chapter 11. Polymers and oligomers of glucose along with dimers of glucose and either galactose, fructose, or glucose are digested efficiently as they travel through the small intestine. Digestion involves the enzymatic cleavage of the oxygen bridges, called glycosidic bonds, that link the hexose units. The released free hexoses, but not the larger sugars or oligosaccharides, are transported across the intestine into the circulation. The processes of enzymatic digestion occur within the lumen of the small intestine under the influence of secreted pancreatic amylase and the abundant constituent oligosaccharidases of the apical membrane of the columnar epithelial enterocytes lining the villi of the small intestine. The fates of the major dietary carbohydrates within the small intestine are summarized in Table 8-1. TABLE 8-1 Summary of Digestion of Dietary Carbohydrate of starch granules varies with each plant species and within different parts of the plant. Starch digestion begins by the action of secreted α-amylase in the lumen of the gastrointestinal tract and is completed by the action of α-glucosidases that are associated with the apical membrane of the intestinal mucosal cells. Thus digestion of starch begins in the mouth by action of salivary α-amylase as food is chewed and mixed with saliva. The action of amylase, however, ceases abruptly after the chewed food mixes with the acidic secretions of the stomach, because amylase activity requires neutral pH. Although the amylase protein is acid labile, the presence of starch in association with the amylase and the protection of amylase within the bolus of food provides some delay in amylase inactivation by the gastric acid (Rosenblum et al., 1988). Pancreatic amylase is secreted in large quantities into the duodenal lumen. Most of the starch component of grains or legumes establishes contact with the pancreatic α-amylases within the polar bulk phase of the intestinal luminal milieu. The concentration of amylase achieved within the duodenal lumen greatly exceeds that required for cleavage of the bonds joining the glucose components of the starches. Indeed, the cleavage of starches to the final oligosaccharide products normally occurs in the uppermost part of the small intestine and is virtually complete by the time the meal reaches the duodenal–jejunal junction (Fogel and Gray, 1973). Furthermore, refined starches are hydrolyzed efficiently to glucose oligomers, even in patients with exocrine pancreatic insufficiency who have amylase levels that are only about 10% of normal (Fogel and Gray, 1973). It is important to consider the structure of the principal dietary starches, because amylase has specificity for only the α(1,4)-linked straight-chain regions of the glucosyl polysaccharide, whereas the most abundant food starches also have α(1,6)-branching links. The simplest starch is the linear and unbranched amylose, which is a polymer of α(1,4)-linked glucosyl units (∼600 glucose residues per molecule). Amylase has maximal specificity for the interior links, and its active site binds five consecutive glucose residues at specific subsites and cleaves between the second and third subsites, as shown in Figure 8-1, to form two smaller polymers. Sequential cleavage eventually leads to the production of pentasaccharides that bind with high affinity at all five of the amylase subsites. The pentasaccharide will be hydrolyzed at the penultimate linkage from the reducing end of the pentasaccharide to release the trisaccharide maltotriose and the disaccharide maltose. Products smaller than the pentasaccharide are not able to bind at all subsites and thus have very low affinity for the amylase active site; hence these smaller oligosaccharides are not readily cleaved by amylase. Overall, the sequential actions of amylase promote the release of maltotriose and maltose as the main final products of luminal amylose digestion. Amylopectin is a more complex form of starch, representing about 80% of dietary polysaccharide. Figure 8-2 depicts the action of amylase on amylopectin to yield the final oligosaccharide products within the distal duodenal lumen. Although its linear segments are similar to those for amylose, amylopectin is branched by virtue of α(1,6) links positioned approximately every 25 residues along the chain. Glycogen, though a minor dietary carbohydrate, has a structure analogous to that of amylopectin and is also digested by amylase. Amylase is unable to cleave the α(1,6) branching link, and the structural angulation created by this linkage inhibits the enzyme from attacking some of the adjacent α(1,4) links. As a consequence, branched oligosaccharides called α-limit dextrins (or α-dextrins), along with maltose and maltotriose, are the final products of amylopectin hydrolysis by α-amylase. Dextrins of average mass of five to six glucose units represent nearly one third the mass of the final breakdown products of amylopectin. Overall, the hydrolytic activities of salivary and pancreatic amylases on amylose and amylopectin result in production of glucose oligosaccharides and disaccharides containing α(1,4) linkages and glucose oligosaccharides containing both α(1,4) and α(1,6) linkages, with only about 4% of the glucose residues being released as free glucose monomers (Yook and Robyt, 2002). The subsequent steps of starch digestion are ensured by activities of the α-glucosidases associated with the apical membrane of the intestinal mucosal cells, as discussed later. Both salivary and pancreatic amylase levels are low at birth but typically reach adult levels before 1 year of age. Expression of pancreatic amylase activity appears to lag behind the expression of other enzymes synthesized in the acinar cells of the pancreas. This has prompted some pediatricians and nutritionists to recommend withholding starch from the diet until about 6 months of age. Nevertheless, clinical symptoms resulting from cereal feeding before 6 months of age are rare (Lebenthal et al., 1983). The apical (brush border) membrane surface of the small intestinal enterocytes is replete with oligosaccharidases. Two of these oligosaccharidases are α-glucosidases that carry out the final steps in starch digestion (Semenza and Auricchio, 1991). Each one of these α-glucosidases, as synthesized, possesses two domains, with each domain containing an independent active site. The enzyme molecules are named for the main activities of the two domains: maltase–glucoamylase and sucrase–isomaltase. Both are attached to the apical membrane by an N-terminal hydrophobic sequence that spans the membrane. Maltase–glucoamylase, isomaltase (α-dextrinase), and sucrase work in a complementary manner to cleave the bonds in the α-dextrins in sequence from the nonreducing end to release free glucose units. As shown in Figure 8-3, at each step in the process, one or more of these enzymes has high specificity for the α-glucosyl link closest to the nonreducing end of specific oligosaccharide products. These glucosidases produce free glucose for transport into the enterocyte. Maltase–glucoamylase has a higher affinity (lower Km) and faster rate of substrate turnover (higher kcat) for hydrolysis of glucose oligomers than does sucrase–isomaltase, and thus it will catalyze a more rapid rate of hydrolysis at low concentrations of glucose oligomers (Table 8-2). However, it appears that maltase–glucoamylase contributes a relatively small fraction of the total glucose oligomer-hydrolyzing activity in humans in vivo. Indeed, sucrase–isomaltase activity in humans is about 20 times that of maltase–glucoamylase activity. Moreover, sucrase–isomaltase is not inhibited by lumenal starch-derived oligosaccharides as is the activity of maltase–glucoamylase in assays performed in vitro (Quezada-Calvillo et al., 2007). Thus sucrase–isomaltase probably contributes most of the α-glucosidase activity when high-starch diets are consumed. TABLE 8-2 Kinetic Constants for α-Glucosidases for Oligosaccharide Hydrolysis ∗Km, Michaelis constant; the substrate concentration at which an enzyme or transporter is half-saturated with substrate. †kcat, First-order rate constant corresponding to the slowest step or steps in the overall catalytic reaction; the number of catalytic cycles the active site undergoes per unit time. In addition to digestion of maltose, as described for the end products of starch breakdown, humans have the capacity to hydrolyze unique linkages present in sucrose, lactose, and trehalose. Kinetic properties of these disaccharidase activities are shown in Table 8-3. These disaccharidases are all associated with the luminal surface of the enterocytes, as illustrated in Figure 8-4. TABLE 8-3 ∗Km, Michaelis constant; the substrate concentration at which an enzyme or transporter is half-saturated with substrate. †kcat, First-order rate constant corresponding to the slowest step or steps in the overall catalytic reaction; the number of catalytic cycles the active site undergoes per unit time. Trehalose [α-D-glucopyranosyl-(1,1′)α′-D-glucopyranoside] is a disaccharide present in small amounts in bacteria, fungi, plants, and invertebrates (Elbein et al., 2003; Richards et al., 2002). Significant food sources of trehalose in modern Western diets include baker’s yeast, brewer’s yeast, cultivated and wild mushrooms, honey, sunflower seeds, sea algae, lobster, shrimp, and processed foods to which trehalose has been added. Trehalose may have constituted a larger source of sugar in the diet of ancient man, and some populations eat a much higher proportion of invertebrates (e.g., insects in which trehalose is the major hemolymph sugar) and fungi (e.g., yeasts, molds, mushrooms, and truffles) as part of their diets and therefore ingest more trehalose than is provided by typical Western diets. Reported concentrations of trehalose from natural sources vary because of experimental conditions, analytical methods, and the life stage of the organism assayed. In organisms, trehalose performs diverse functions that include energy storage and transport as well as protection against extreme temperatures or desiccation (Schiraldi et al., 2002). In humans the oligosaccharidases and disaccharidases are present in the gut before birth and are expressed throughout life. In general, levels of these enzymes are reduced in subjects who have fasted or are receiving parenteral nutrition, and levels are increased in subjects fed a carbohydrate-rich diet or in patients with uncontrolled diabetes. In most other mammals the lactase–phlorizin hydrolase is expressed from birth to weaning, and the other oligosaccharidases appear during the transition from maternal milk to starch-based diets. Lactase–phlorizin hydrolase in the intestinal mucosa of humans reaches maximal activity at an early postnatal age and then declines before adulthood in most people (Simon-Assmann et al., 1986). NF-LPH1 is a transcription factor that is present exclusively in intestinal epithelium and is thought to regulate expression of the lactase–phlorizin hydrolase gene. NF-LPH1 declines simultaneously with lactase–phlorizin hydrolase activity during the postweaning period. Thus a reduction in the expression of NF-LPH1 might be the cause of the decline in lactase activity that occurs before adulthood in most humans (Kuokkanen et al., 2003; Troelsen et al., 2003). Expression of oligosaccharidase and disaccharidase activities at the cellular level is tightly linked to enterocyte differentiation (Boudreau et al., 2002; Troelsen et al., 1997). For example, immature enterocytes developing from stem cells in crypts of the intestinal mucosa do not have sucrase–isomaltase activity. As enterocytes traverse to the crypt–villus junction, sucrase–isomaltase messenger RNA (mRNA) and sucrose–isomaltase activity are observed to increase. The level of sucrase–isomaltase mRNA reaches its peak when enterocytes are located in the lower and mid villus region and then progressively decreases as these cells move toward the tip (Traber et al., 1992). Expression of other disaccharidase and oligosaccharidase activities follows a similar pattern. The posttranslational processing of newly synthesized oligosaccharidases and disaccharidases has been the subject of a number of studies. The general pathways, summarized in Figure 8-5, are similar for lactase–phlorizin hydrolase, sucrase–isomaltase, and maltase–glucoamylase, although each has some specific and unique characteristics (Weisz and Rodriguez-Boulan, 2009). Trehalase expression and processing has little similarity to that of the other disaccharidases. All of these oligosaccharidases and disaccharidases are synthesized in the enterocyte where they are processed in the endoplasmic reticulum (ER) and Golgi apparatus with final vectorial transport to the apical membrane. Lactase–phlorizin hydrolase, sucrase–isomaltase, and maltase–glucoamylase all undergo extensive N– and O-glycosylation in the ER and Golgi apparatus, but trehalase has only N-glycosylation sites. The orientation of these enzymes in the membrane is such that the oligosaccharidase domains, including the active catalytic sites, are on the luminal side of the apical membrane and thus available for efficient cleavage of the luminal substrates. Maltase–isomaltase and sucrase–isomaltase are both anchored in the membrane by an N-terminal hydrophobic sequence, whereas lactase–phlorizin hydrolase is inserted into the plasma membrane by its C-terminus, as illustrated in Figure 8-4. In contrast to the other disaccharidases, the C-terminus of trehalase is anchored to the plasma membrane via a glycosylphosphatidylinositol (GPI) anchor. Proteolysis is involved in production of the final mature forms of sucrase–isomaltase and lactase–phlorizin hydrolase, whereas no proteolytic processing has been documented to occur for human maltase–glucoamylase. The human lactase–phlorizin hydrolase gene encodes a polypeptide of four domains, but two of these domains are eliminated during the maturation process to render a mature protein that has two domains containing one potential active site each. Proteolytic processing occurs intracellularly, and proteolytic trimming by pancreatic proteases in the small intestine occurs at a second site after the enzyme has been transferred to the plasma membrane. The N-terminal domains of lactase–phlorizin hydrolase, which are intracellularly cleaved to yield the active brush border enzyme, are required as a chaperone for exit of the mature polypeptide from the ER. The phlorizin hydrolase domain appears to serve an additional chaperone role and is also required for correct folding and trafficking of the lactase domain out of the ER (Behrendt et al., 2010). The O-glycosylation that is added in the Golgi apparatus is required for sorting of lactase−phlorizin hydrolase to the luminal membrane. Sucrase–isomaltase is transported via the vesicular system to the brush border membrane of enterocytes where it is then subjected to extracellular processing by pancreatic proteolytic enzymes present in the intestinal lumen. The molecule is cleaved to generate a membrane-bound N-terminal isomaltase subunit and a free C-terminal sucrase subunit, which remains associated with the N-terminal isomaltase domain through noncovalent interactions. The C-terminal domain of sucrase–isomaltase appears to act as an intramolecular chaperone for the isomaltase domain and is required for the protein to exit the ER and for subsequent proteolytic processing of the enzyme. In the absence of the sucrase domain, isomaltase remains associated with the ER through its association with the folding chaperone calnexin and is not processed properly (Jacob et al., 2002).
Digestion and Absorption of Carbohydrate
Digestion of Starches
FOOD SOURCE
% OF DIETARY CARBOHYDRATE
PRODUCTS OF LUMINAL HYDROLYSIS
PRODUCTS OF BRUSH BORDER MEMBRANE HYDROLYSIS
Starches (amylose, amylopectin)
60 to 70
Maltose, maltotriose, and α-dextrins
Glucose
Lactose
0 to 10
None
Glucose and galactose
Sucrose
30
None
Glucose and fructose
Luminal Digestion of Starches
Digestion of Starches by Soluble α-Amylase
Differences in Structure and Digestion of Amylose and Amylopectin
Regulation of Amylase Secretion
Completion of Starch Digestion by Integral Membrane Oligosaccharidases
Roles of Maltase–Glucoamylase and Sucrase–Isomaltase in Starch Digestion
Contributions of Maltase–Glucoamylase and Sucrase–Isomaltase to Oligosaccharide Hydrolysis
Glucose Residues
Maltase–Glucoamylase
Sucrase–Isomaltase
N
Km∗ (mmol/L)
kcat† (sec−1)
Km (mmol/L)
kcat (sec−1)
2
2.1
56
14
3.2
3
1.1
149
21
3.1
4
0.4
78
24
3.5
5
0.4
63
61
3.4
6
0.7
70
57
2.1
7
1.0
80
120
2.2
Digestion of Dietary Disaccharides
ENZYME
PRINCIPAL SUBSTRATE
Km∗ (mmol/L)
kcat† (sec−1)
α-GLUCOSIDASES
Sucrase
Sucrose
18
120
Trehalase
Trehalose
3
20
β-GALACTOSIDASE
Lactase
Lactose
2
4
Trehalose Digestion by Trehalase
Expression and Processing of the Oligosaccharidases and Disaccharidases
Effect of Developmental Stage and Diet on Saccharidase Expression
Crypt–Villus Expression of Saccharidases
Posttranslational Processing of Saccharidases
Stay updated, free articles. Join our Telegram channel
Full access? Get Clinical Tree
Digestion and Absorption of Carbohydrate
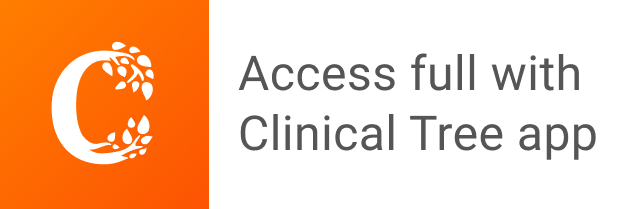