Defenses Against Oxidative Stress1
Dean P. Jones
1Abbreviations: AMD, age-related macular degeneration; ATP, adenosine triphosphate; Cd, cadmium; CYP, cytochrome P-450; Cys, cysteine; CySSG, cysteine-glutathione disulfide; DRI, dietary referintake; ER, endoplasmic reticulum; Fe2+, ferrous iron; Fe3+, feriron; G6P, glucose 6-phosphate; GGT, γ-glutamyltransferase; GPX, glutathione peroxidase; GSH, glutathione; GSSG, glutathione disulfide; GST, glutathione transfer; H2O2, hydrogen peroxide; HNE, 4-hydroxynonenal; Met, methio; NAD+, oxidized nicotinamide adenine dinucleotide; NADH, reduced nicotinamide adenine dinucleotide; NADP+, oxidized nicotinamide adenine dinucleotide phosphate; NADPH, nicotinamide adenine dinucleotide phosphate; NO·, nitric oxide; NOS, nitric oxide synthase; Nox, nitric oxide; NOS, nitric oxide synthase; ·OH, hydroxyl radical; PDI, protein disulfide isomerase; Prx, peroxiredoxin; PUFA, polyunsaturated fatty acid; RNS, reactive nitrogen species; ROS, reactive oxygen species; Sec, selenocysteine; SOD, superoxide dismutase; Trx, thioredoxin; UV, ultraviolet.
OVERVIEW
Antioxidant defenses against oxidative stress have a rich history of scientific investigation, influenced by medicine, public health, commerce, and politics. Since 2000, considerable change in the scientific focus has occurred as results from large-scale, double-blind interventional trials with free radical scavenging antioxidants became available to show little to no benefit of these supplements to protect against human disease. The results of these antioxidant trials do not invalidate the substantial data associating oxidative stress with disease and antioxidants with protection against oxidative stress. Instead, the results indicate that oxidative stress is not adequately described as a simple imbalance between pro-oxidants and antioxidants.
This chapter surveys the sources and types of oxidative stress and the multiple systems that interact to maintain physiologic function and protect against disease. This is an active area of nutrition research, with important unknowns, uncertainty, and controversy. In addition to extensively studied mechanisms of oxidative damage to macromolecules, contemporary research emphasizes the importance of nutrition to support redox signaling. Redox signaling refers to pathways of cell and subcellular communication that involves oxidants; the associated signaling proteins are key sites of oxidative disruption in human diseases. Diet and nutrition are central to the function of these signaling systems, directly by maintaining the redox signaling components (e.g., enzymes, transporters, transcription factors) and indirectly by optimizing gene expression and epigenetic control of protective and repair systems.
Despite controversies and ongoing investigations, extensive research supports modern nutrition policies emphasizing adequacy of intake of the antioxidant vitamins C and E and the antioxidant mineral selenium as defined for their dietary reference intake (DRI) values. Zinc (Zn2+), several vitamins (e.g., vitamin D and B vitamins), and some amino acids (methionine [Met], cysteine [Cys], glutamine) are important to maintain antioxidant functions dependent on glutathione (GSH). These indirect antioxidant functions can be important under some conditions and are addressed within DRIs, but they are not primary criteria for DRI values. At the same time,
nutrition policies recognize a need to avoid excesses of some nutrients because of associated risks (e.g., vitamin E, selenium, iron in postmenopausal women and men, copper, and, in some cancer-prone populations, β-carotene). Ongoing research on nutrition and defenses against oxidative stress increasingly employs approaches that provide better spatial and temporal resolution of oxidative reactions within cells, focus on nonradical mechanisms, and integrate redox systems biology of the complete genome, epigenome, proteome, and metabolome.
nutrition policies recognize a need to avoid excesses of some nutrients because of associated risks (e.g., vitamin E, selenium, iron in postmenopausal women and men, copper, and, in some cancer-prone populations, β-carotene). Ongoing research on nutrition and defenses against oxidative stress increasingly employs approaches that provide better spatial and temporal resolution of oxidative reactions within cells, focus on nonradical mechanisms, and integrate redox systems biology of the complete genome, epigenome, proteome, and metabolome.
DEFINITION OF OXIDATIVE STRESS
Oxidative stress is defined as an imbalance in pro-oxidant and antioxidant reactions that causes macromolecular damage or disrupts biologic redox signaling and control (1). This encompasses a broad spectrum of processes affecting the health of biologic systems, as depicted in Figure 46.1. All these processes involve electron transfer, or “redox,” reactions, in which loss of one or more electrons (termed oxidation) occurs from a donor chemical, and gain of one or more electrons (termed reduction) occurs by an acceptor chemical. Conservation of matter requires that these processes be coupled (i.e., whenever something is oxidized, something else becomes reduced). The bias in use of oxidative stress, instead of reductive stress, is derived from life in an aerobic environment, where the carbon-, hydrogen-, nitrogen- and sulfurcontaining chemicals are oxidized while O2 serves as the electron acceptor, ultimately being reduced to water.
Pro-oxidants and Antioxidants
Pro-oxidants are agents that stimulate aberrant electron transfer in biologic systems and cause oxidative stress; these include both free radical oxidants and nonradical oxidant agents that initiate radical reactions, oxidize biologic components, or interfere with normal reductive and antioxidant functions. Free radicals (or more simply, radicals) are organic molecules or ions with an unpaired electron that are often reactive and function as pro-oxidants. Radicals support a unique chemistry in which chain reactions occur, such as those involved in polymerization of plastics. Nonradical oxidants are chemicals that participate in oxidation reactions not involving radical mechanisms. Antioxidants (Fig. 46.2) are agents that function at low concentrations to stop oxidative stress. These include radical-scavenging chemicals that accept or donate electrons to terminate radical chain reactions (see later) and chemicals and enzyme systems that eliminate or protect against oxidants, some of which are listed in Figure 46.3.
The term antioxidant is loosely defined and also includes agents that inactivate radical initiation and catalysis, such as metal ion chelators, agents that block radiation-induced
oxidation, and agents that counter oxidation of a substance with reduction. The term includes agents that protect against radical and nonradical oxidative mechanisms, and more specific terms such as “radical-scavenging antioxidant” or “thiol antioxidant” are used to discriminate these. The reactions of pro-oxidants and antioxidants have been extensively studied and provide a foundation to understand the biochemistry and biology of oxidative stress.
oxidation, and agents that counter oxidation of a substance with reduction. The term includes agents that protect against radical and nonradical oxidative mechanisms, and more specific terms such as “radical-scavenging antioxidant” or “thiol antioxidant” are used to discriminate these. The reactions of pro-oxidants and antioxidants have been extensively studied and provide a foundation to understand the biochemistry and biology of oxidative stress.
Challenges to Define Antioxidant Requirements
Knowledge about the chemistry of oxidative reactions is derived from studies with purified chemical systems. Extrapolation of this chemistry to biology and nutrition remains challenging because the simple models do not effectively describe complex systems. For most vitamins and minerals, support of specific proteins and biologic functions allows nutritional needs to be considered in terms of the amounts needed for those respective functions. Because of the limited numbers of interaction sites on proteins, an amount of nutrient that is sufficient to saturate the sites or activities can be determined. However, such criteria do not exist for chemical reactions with high rate constants, such as those involved in free radical reactions associated with oxidative stress. For these reactions, one must extrapolate data from homogeneous chemical systems to nonhomogeneous biologic systems.
![]() Fig. 46.3. Pro-oxidant radicals and nonradical oxidants in biologic systems. (See text for descriptions) |
Within a compartment such as mitochondria or chromatin, the rate of reaction at a specific subcellular site is critical. Antioxidants that block radical reactions are consumed at such sites, with the result that the subcellular compartment becomes a “sink” for the antioxidant. Diffusion of an antioxidant to a sink varies as a function of the concentration difference (ΔC) between the source (e.g., blood) and the sink, according to the Fick equation (flux = diffusion coefficient × ΔC). In principle, there is no upper limit to the amount of antioxidant that could be beneficial. This is fundamentally different than for vitamins and minerals that saturate protein-dependent systems, and it represents an ongoing challenge to define antioxidant requirements. At the same time, recognition of this problem has led to an effort to develop more effective antioxidants by improving delivery to specific sites, such as design of specific mitochondrial antioxidants based on transport characteristics (2). Furthermore, beyond the problem of defining requirements for delivery to specific subcellular sites, defining antioxidant requirements is limited by overlapping and redundant activities. Multiple antioxidants block the same oxidative reactions. Thus, even though an overall antioxidant capacity may be needed, assignment of that requirement to an individual chemical is not possible.
Vitamin C and E Are the Only Antioxidants with Dietary Reference Intakes
Many naturally occurring antioxidant chemicals block free radical reactions, but only two, vitamins C (ascorbate) and E (α-tocopherol), have been found to have sufficient
specificity for elimination of disease to warrant inclusion as vitamins (see the chapters on vitamin C and vitamin E). The DRI for ascorbate is based in part on the amount needed to support specific enzyme activities. Ascorbate does not show the characteristic of the hypothetic benefit of increased intake because it is water soluble, and its reabsorption by the kidneys is saturable. As a consequence, ascorbate ingested in excess of about 250 mg/day is directly lost in the urine. Establishment of DRI values for the fatsoluble α-tocopherol is less direct, but it similarly includes consideration of a specific α-tocopherol-binding protein in liver. Other antioxidants support the same types of radicalscavenging activities as vitamin C and E but do not have the same biochemical evidence to define a requirement. As indicated earlier, other chemicals have overlapping and redundant antioxidant activities so that requirements are difficult to establish and justify scientifically.
specificity for elimination of disease to warrant inclusion as vitamins (see the chapters on vitamin C and vitamin E). The DRI for ascorbate is based in part on the amount needed to support specific enzyme activities. Ascorbate does not show the characteristic of the hypothetic benefit of increased intake because it is water soluble, and its reabsorption by the kidneys is saturable. As a consequence, ascorbate ingested in excess of about 250 mg/day is directly lost in the urine. Establishment of DRI values for the fatsoluble α-tocopherol is less direct, but it similarly includes consideration of a specific α-tocopherol-binding protein in liver. Other antioxidants support the same types of radicalscavenging activities as vitamin C and E but do not have the same biochemical evidence to define a requirement. As indicated earlier, other chemicals have overlapping and redundant antioxidant activities so that requirements are difficult to establish and justify scientifically.
Contemporary Refinement in Definition
The contemporary definition of oxidative stress has been refined based on research findings since 2000 (1, 3, 4, 5). At this time, evidence became available to show that the principal thiol-disulfide systems controlling the oxidationreduction states of proteins are not in a near-equilibrium state but rather are kinetically limited (6). Coupled with rapidly developing knowledge of redox-signaling nicotinamide adenine dinucleotide phosphate (NADPH) oxidases, this discovery provided a framework to consider thiol redox circuits as a global system for regulation of cell and organ system function (7, 8). At the same time, results of large-scale, double-blind interventional studies with radical-scavenging antioxidants also began to appear in the literature (9, 10, 11, 12, 13, 14, 15, 16). These studies showed that supplementation with antioxidants (i.e., shifting the “balance” toward protection) had little or no health benefit in humans. Consequently, the original definition of oxidative stress has been qualified in the sense that an imbalance is now considered only in terms of specific reactions or pathways and not in terms of a “global” imbalance. In other words, in the current conceptualization, an imbalance in a specific reaction or pathway can cause damage without an overall imbalance in the system, and a change in overall balance of pro-oxidants and antioxidants can occur without causing damage.
The modern view of oxidative stress is also affected by the development of “-omic” technologies and systems biology. Older criteria of oxidative stress were limited to gross measures, such as cell death (17) or macromolecular damage, including lipid peroxidation (18), global protein carbonylation (19), or DNA damage (20). Modern methods support measures of expression of specific genes (21), modification of specific lipids (22), oxidation of specific proteins (23), and global effects on metabolic pathways (24, 25). These methods are enabling detailed understanding of the nutritional contributions to both radical and nonradical mechanisms of oxidative stress.
SPECTRUM OF OXIDATIVE STRESS
The spectrum of oxidative stress can be considered in terms of oxidants and pro-oxidants directly from the diet and environment (see Fig. 46.1A), oxidants generated endogenously with failure of endogenous protective systems (see Fig. 46.1B), and more subtle disruption of redox signaling and control mechanisms (see Fig. 46.1C). For some of these, nutrition provides limited to no protection. For others, antioxidant defenses are clearly important and are affected by nutrition.
Environmental Causes of Oxidative Stress
Physical forces in the environment often represent an unavoidable source of oxidative stress. These forces are important in nutrition because they can affect the quality of food and nutritional supplements as well as have direct effects on human health. Oxidation during food storage and preparation adversely affects palatability and nutrient content. In general, this oxidation occurs by the same mechanisms described here, is managed within the food industries to maximize product shelf life, and is incorporated in nutritional recommendations and food guides to account for such losses. Consequently, only the biologic effects of oxidative stress are addressed here.
Visible light can cause oxidative damage, but only the more intense blue light has been linked to disease. Blue light damages the retinal pigment epithelial cells in the sensory retina and contributes to age-related macular degeneration (AMD) (26). Ultraviolet (UV) light from the sun causes more extensive oxidative stress, such as oxidative damage to the skin, resulting in sunburn and skin cancers (27, 28). The evolution of humans as diurnal rather than nocturnal mammals, with relatively poor protection by hair, has resulted in nutritional requirements that are distinct from requirements for other species. Ionizing radiation causes oxidative DNA damage and contributes to leukemias and other cancers (29, 30). Sound waves cause oxidative damage in lithotripsy of kidney stones and damage cochlear cells in hearing loss (31, 32). Heat increases oxidative reactions with resulting oxidative damage in thermal injury. Thus, exposures in the physical world include unavoidable causes of oxidative stress.
Few nutritional means are available to protect against these physical forces, but some exceptions exist. Some carotenoids accumulate in the retina and protect against light-induced damage. Lutein and zeaxanthin, in particular, accumulate regionally in the macula and are currently being studied for protection against AMD at doses of 10 mg/day lutein and 2 mg/day zeaxanthin (26) in a double-blind randomized trial—the Age-Related Eye Disease Study-2 (AREDS2). Carotenoids function as intraocular filters to capture and dissipate light energy and scavenge singlet oxygen, a reactive form of molecular oxygen generated by photochemical activation (33). UV radiation is most effectively blocked by melanin, a natural
polymer derived from tyrosine oxidation. Melanin production is deficient in albinism and is partially blocked by high phenylalanine in uncontrolled phenylketonuria. Pigmentation color is partially determined by Cys through a reaction that limits polymerization and results in reddish, rather than darker, pigments. For none of these reactions is nutrition very useful to enhance protection from UV radiation. Conversely, regular consumption of cocoa has been shown to protect against erythema from UV radiation, perhaps because of activation of endogenous antioxidant systems (34). Additionally, sulfur amino acids, lysine, zinc, vitamin C, and polyunsaturated fatty acids (PUFAs) used for inflammatory signaling may provide some benefit in the repair processes. Avoidance of sun exposure and use of commercial sunscreens are popular means to protect against UV light injury, but these limit natural synthesis of vitamin D in the skin.
polymer derived from tyrosine oxidation. Melanin production is deficient in albinism and is partially blocked by high phenylalanine in uncontrolled phenylketonuria. Pigmentation color is partially determined by Cys through a reaction that limits polymerization and results in reddish, rather than darker, pigments. For none of these reactions is nutrition very useful to enhance protection from UV radiation. Conversely, regular consumption of cocoa has been shown to protect against erythema from UV radiation, perhaps because of activation of endogenous antioxidant systems (34). Additionally, sulfur amino acids, lysine, zinc, vitamin C, and polyunsaturated fatty acids (PUFAs) used for inflammatory signaling may provide some benefit in the repair processes. Avoidance of sun exposure and use of commercial sunscreens are popular means to protect against UV light injury, but these limit natural synthesis of vitamin D in the skin.
Ionizing radiation from radioactive decay is similar to UV light in that nutrition is important in inflammatory signaling and repair but not by providing an ability to filter the radiation. For certain radiation exposures, however, supplemental iodine is thought to be beneficial by displacing and facilitating excretion of radioactive iodine. Precursors of GSH have been studied as means to protect against side effects of radiation therapy in cancer; utility is limited because decreased tumoricidal activity accompanies protection of normal tissues. Consequently, common sense and avoidance are most important when confronting oxidative stress from physical exposures because of the limited benefits beyond sound nutritional strategies for health.
Direct-Acting Inorganic Chemicals and Xenobiotics
In addition to physical forces, direct-acting inorganic chemicals cause oxidative stress, especially affecting the respiratory and gastrointestinal systems. Atmospheric pollution contains certain reactive oxygen species (ROSs) and reactive nitrogen species (RNSs) that contribute to oxidative damage in the airways (35, 36). ROSs are oxygen-containing chemicals that are reactive with organic molecules and include superoxide anion radical, hydrogen peroxide (H2O2), lipid hydroperoxides, ozone, and related oxygen-centered radicals (Fig. 46.4). RNSs include nitric oxide (NO·), peroxynitrite, and other oxides of nitrogen. The terms ROS and RNS are commonly used because the chemistry is too complex and the analytic methods are insufficient to identify specific reactive species.
Many transition metals catalyze electron transfer and also are important environmental sources of oxidative stress. Environmental dispersal of oxidative transition metals such as iron (37) and cadmium (Cd) (38, 39, 40) can cause acute toxicities. Cd is especially important as a contaminant in the diet because it is a common pollutant associated with industrialization and natural recycling of wastes as fertilizers. Cd was found to be increasing in some agricultural areas of southern Sweden at a rate of 1% per year. The biologic half-life of Cd in humans is about 20 years because of limited excretion. Pharmaceuticals (41), drugs of abuse, and chemicals from commercial products and industrial wastes (42, 43) also provide exogenous sources of oxidative stress. Ames (44) emphasized that natural toxicants in food pose a much greater risk than do manufactured sources. Although this may be generally true, the apparent increase in food borne toxicants, which cannot be compensated for by better nutrition, indicates an important need for nutrition and food scientists to limit sources of such contaminants.
Endogenous Superoxide and Peroxide Production
The most actively studied areas of nutrition and oxidative stress have involved endogenous oxidant generation and the failure of endogenous antioxidant defenses (see Fig. 46.1). This includes damaging processes that result from central oxidative metabolism processes in cells. The major macromolecules of cells (protein, nucleic acids, lipids, carbohydrates) are composed mostly of carbon, hydrogen, oxygen, nitrogen, phosphorus, and sulfur—elements that form stable chemical structures in which pairs of electrons are shared between elemental nuclei. The interconversion of these biochemicals mostly involves conservation of the pairs of electrons, with hydride ion transfer via reduced nicotinamide adenine dinucleotide/oxidized nicotinamide adenine dinucleotide (NADH/NAD+) or NADH/oxidized nicotinamide adenine dinucleotide phosphate (NADP+) (see Fig. 46.4) providing a common mechanism for 2-electron (2-e–) transfer. These electron transfers occur in highly specific reactions, many of which have relatively high rates to support critical needs for energy metabolism, anabolism and repair, detoxification, and elimination of wastes.
Oxidant Production in Energy Metabolism
These 2-e–-transfer reactions linked to NADH/NAD+ and NADPH/NADP+ are connected to one-electron (1-e–) transfer reactions in metabolic pathways through chemical structures that exist in interconvertible fully oxidized, 1-e–-reduced and 2-e–-reduced forms (see Fig. 46.4). These include hydroquinone/semiquinone/ quinone and flavoprotein systems, with coenzyme Q providing an example of the former and the flavin mononucleotide (FMN) of mitochondrial NADH dehydrogenase providing an example of the latter. Living organisms use 1-electron chemistry in central energy capture and transfer reactions of photosynthesis and mitochondrial respiration. These 1-e– transfers are physically contained within special membrane structures of chloroplasts and mitochondria. These features support the oxidation of macromolecular energy sources (fat, carbohydrate, and protein) through 2-e– transfers with coupling to 1-e– transfer pathways for production of adenosine triphosphate (ATP). The 1-e– systems of mitochondria and chloroplasts are important in oxidative stress because these systems have very high electron transfer rates; are sensitive to visible and UV light, trace metals, oxidants, and reactive electrophiles; and cause extensive destruction to other biologic components.
Photosynthetic plants are rich sources of radical scavenging antioxidants, likely because of their need to control radical injury from sunlight while using the light-derived energy to drive photosynthesis and O2 production. In contrast, mitochondria have high rates of electron transfer and require O2 for ATP production. In both chloroplasts and mitochondria, damaged or malfunctioning electron transfer pathways represent a major source of endogenous oxidative stress.
Disruption of mitochondrial electron transfer has been extensively studied as a contributing mechanism in aging and disease. Damaged mitochondria have increased rates of 1-e– transfer O2 to produce the radical, superoxide anion (O2-·). Hundreds of studies have shown that disruption of mitochondrial function, by many experimental means, results in injury and disease symptoms. Copper deficiency results in aberrant megamitochondria (45). The anticancer drug doxorubicin and antiretroviral drugs used for human immunodeficiency virus (HIV) infection treatment cause mitochondrial damage (46, 47). Such conditions often damage mitochondrial DNA, and increase ROS generation, so such conditions can be self-perpetuating and autocatalytic in destruction of mitochondria. However, evidence that one can protect against mitochondrial oxidative stress by nutritional or dietary antioxidant supplementation in humans is limited.
Mitochondria have multiple defense systems to protect against excessive oxidant generation, and these may be more critically dependent on the adequacy of precursors for NADPH supply than on supplementation with radical scavengers. Endogenous defenses include the electron transfer intermediate, coenzyme Q (48), which exists in the mitochondrial inner membrane in a stable, radical form (semiquinone) that protects against radical reactions. Mitochondria also contain specific GSH and thioredoxin (Trx) systems (49, 50). Pharmacologic forms of coenzyme Q show some promise of benefit in protecting mitochondria, but nutritional forms of coenzyme Q do not appear to be effectively delivered to mitochondrial inner membranes. Both GSH and Trx systems depend on adequate dietary selenium and riboflavin, although excesses of these are considered likely to be harmful, rather than to enhance activity further.
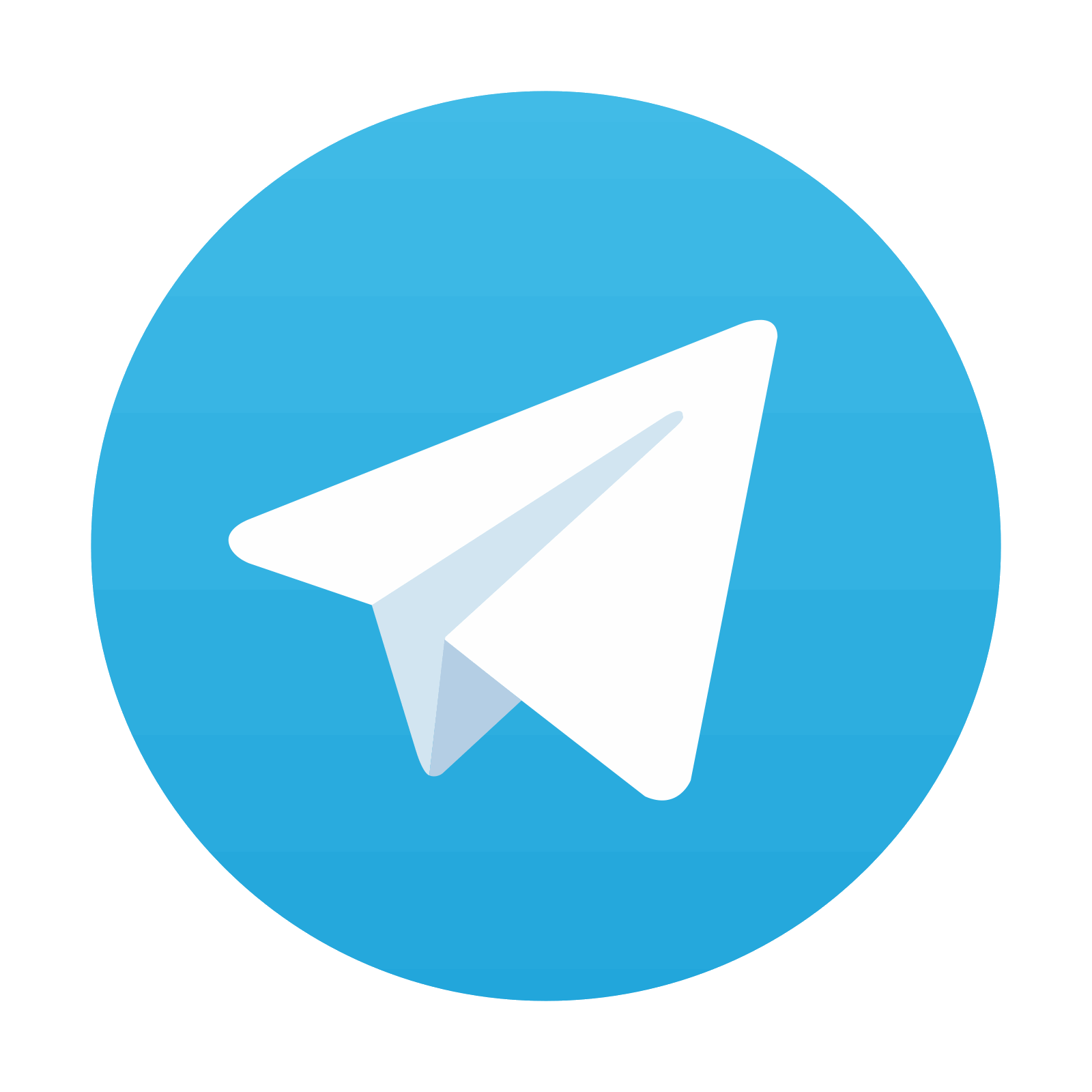
Stay updated, free articles. Join our Telegram channel
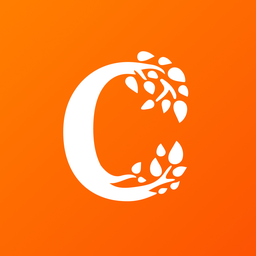
Full access? Get Clinical Tree
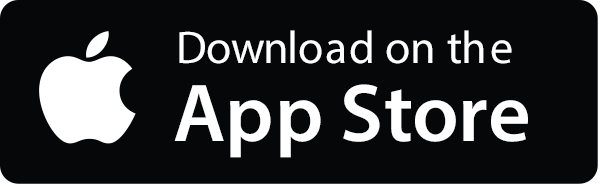
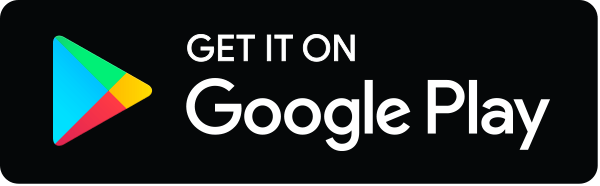
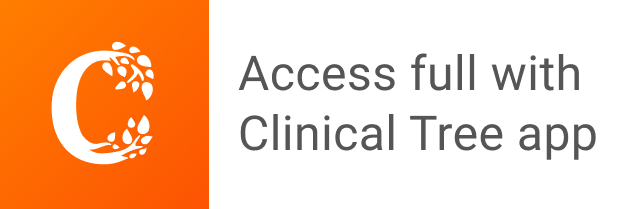