Chapter 15 Anil Zechariah1,2,3; Donald G. Welsh1,2,3,* 1 Hotchkiss Brain Institute, University of Calgary, Calgary, Alberta, Canada TRP channels participate in cation regulation and play crucial roles in a range of vascular functions. In the cerebral circulation, multiple members of this channel superfamily have been reported to be involved with smooth muscle cell proliferation, the development of myogenic tone, and in the regulation of blood flow. TRP channel subunits also influence endothelial permeability, vessel formation, remodeling, and arterial dilation. In this chapter, the authors will briefly outline the key TRP channel subunits, emphasizing on their role in the cerebral circulation. Having defined the key subunits and their role in vascular function/development, the authors will subsequently discuss the connection to cerebrovascular diseases. Of particular interest are the TRP channels that play a role in the development of hypertension and stroke injury. Transient receptor potential (TRP) channels are a large family of cation-specific ion channels that have acquired immense attention, due to their involvement with a variety of cellular functions. TRP multigene family sequence is conserved, both in vertebrates and invertebrates [1,2] and member proteins are classified in subfamilies named TRPC (canonical), TRPV (vanilloid), TRPM (melastatin), TRPA (ankyrin), TRPML (mucolipin), TRPP (polycystin), and TRPN (NOMPC-like; Figure 15.1). Among the subfamilies, 28 members are expressed in vertebrates (with the exception of TRPN) of which 27 are found in humans (excluding TRPC2, which is encoded by a pseudogene) [1,2] (Figure 15.1). TRP channels are expressed in a range of different human cell types and are tied to a variety of cellular processes ranging from stimuli sensation to ion homeostasis [3]. In this chapter, we plan to provide an overview of TRP channels in humans, emphasizing on the function and dysfunction of the cerebral circulatory system. The TRPC subfamily consists of six members (TRPC1, TRPC3, TRPC4, TRPC5, TRPC6, and TRPC7), which are robustly expressed in the human brain. In regard to the vasculature, TRPC1 displays a ubiquitous distribution pattern, whereas C3, C4, and C6 are expressed in vascular smooth muscle cells and C4 in the endothelium (Figure 15.1). These channels are engaged in brain development, cell differentiation, mechanosensation, arterial tone control, smooth muscle cell proliferation, and angiogenesis (for detailed review, see Ref. [2]). The human TRPV subfamily also contains six members (TRPV1, TRPV2, TRPV3, TRPV4, TRPV5, and TRPV6), all of them being represented in the brain tissue (Figure 15.1). V2 subunit was found in smooth muscle cells and V3, V4 in the vascular endothelium, playing key roles in thermosensation, mechano/osmolarity sensation, calcium reabsorption, and synaptic adaptation [2,3]. In humans, there are eight members of the TRPM subfamily (TRPM1, TRPM2, TRPM3, TRPM4, TRPM5, TRPM6, TRPM7, and TRPM8) among which M7 is distributed ubiquitously, whereas M2, M3, and M5 variants are expressed in the brain and M4 and M8 are also found in the smooth muscle cell layer [2] (Figure 15.1). These subunits are thought to play an integral part in myogenic tone development, apoptosis, cell cycle control, thermosensation, and Mg2 + homeostasis [2,3]. The TRPA subfamily is comprised of a sole member (TRPA1) (Figure 15.1), typically associated with thermosensation, olfactory responses, and endothelium-dependent dilation [2,3]. The TRPML subunit consists of TRPML1, TRPML2, and TRPML3 (Figure 15.1); however, there is no evidence of TRPML expression in the resistance vasculature of the brain [2,3]. On the other hand all three members of the TRPP subfamily (TRPP2, TRPP3, and TRPP5) are present in arteries. These subunits have been linked to pressure and flow sensing along with the maintenance of vessel wall integrity [2,3]. TRP channels are associated with a variety of physiological processes in humans including signal transmission, nociception, thermosensation, osmolarity regulation, and arterial tone development [4]. TRP channel activity is triggered by changes in cellular temperature, pressure, chemical agents, osmolarity, and pH [4,5] and by naturally occurring spices, herbs, toxins, and venoms [4,6]. Changes in intercellular calcium (Ca2 +), induced by the activation of G-protein coupled receptors, phosphatidylinositol 4,5-bisphosphate, diacylglycerol (DAG), adenosine triphosphate (ATP), and calmodulin are also known to influence TRP channel activities [4,7]. In addition, interactions of TRP channels with intercellular proteins forming “channelosomes” are now believed to effect its trafficking, positioning, and activity [4,8,9]. Consequently, mutations in TRP channel encoding genes may be responsible for several diseases of the cerebrovascular and cardiovascular systems [4]. TRPC3, TRPC5, TRPC6, TRPM2, TRPM4, TRPM7, and TRPML1 have been implicated in CNS disorders, whereas TRPC1, TRPC3, TRPC6, and TRPM4 have been linked to cardiovascular abnormalities [4]. Although human genetic studies have demonstrated the role of TRP gene mutations in at least 12 hereditary diseases (e.g., polycystic kidney disease, hereditary motor and sensory neuropathy, spinal muscular atrophy, and progressive familial heart block), transgenic and knockout animal experiments, on the other hand, have revealed the role of TRP channels in the development of disease pathologies [4,10]. By targeting TRP channels, novel treatment strategies could be deducted for these so-called TRP channelopathies, a rapidly expanding category of diseases. TRP channel targeting molecules have been developed and tested clinically in recent years with minimum success [11]. For example, TRPV1 antagonist underwent clinical trials for the treatment of inflammatory, visceral, and neuropathic pain; however, this resulted in adverse side effects such as hyperthermia and impaired heat sensation in patients [11]. Vascular smooth muscle cells (VSMCs) account for the majority of blood vessel wall composition and hence are widely distributed, playing major roles in circulatory, respiratory, gastrointestinal, and urogenital systems [12,13]. Although blood flow regulation by contracting and relaxing the blood vessel remains the primary function of VSMCs, they have been implicated in many other physiological functions such as proliferation and migration, production of cytokines/chemokines/matrix proteins/growth factors/cell surface adhesion molecules, and in antigen presentation [12–17]. Smooth muscle activity, in large part, is determined by the changes in Ca2 + concentration, a process dependent on Ca2 + entry from the extracellular space through ion channels and on the Ca2 + release from the intracellular calcium stores [12]. Extracellular Ca2 + entry is facilitated by voltage-operated calcium channels (L-type and T-type) and voltage-independent calcium channels (receptor-operated channels, store-operated channels (SOC), and stretch-activated channels). TRP channels have been reported to be a key component of the voltage-independent calcium channels in the SMCs; their dysfunction is responsible for vascular pathologies ranging from arterial hypertension and arthrosclerosis, to stroke and cardiovascular diseases [12,18]. TRP channels have been implicated in the proliferation and migration of SMCs following vascular injury in humans. Smooth muscle proliferation requires a shift in the smooth muscle profile from a contractile phenotype to a synthetic phenotype, which is facilitated by transcriptional changes in regulatory proteins and is associated with the changes in the expression of ion channels [19]. Of note is the observation that the shift in smooth muscle phenotype also contributes to atherosclerosis and the formation of hypertensive microvessels [19]. Among TRP subtypes, TRPC (especially TRPC1, TRPC4, and TRPC5) are all known to be key players in smooth muscle proliferation [19]. Moreover, TRPC up-regulation was observed in patients with idiopathic pulmonary arterial hypertension, characterized by the abnormal increase of SMCs in the pulmonary artery [20]. Many TRP channel subtypes are linked with the regulation of contractile function in smooth muscle cells. TRPC1 is shown to be an essential component of SOC in inducing vessel contractions, whereas, on the other hand, vessel contraction resulting from TRPC6-activated DAG appears to work independent of store depletion [21,22]. TRPC3 is known to be involved with the regulation of blood flow in cerebral vessels, the suppression of which deceased constriction response to uridine triphosphate [23]. Conversely, TRPV4 has been shown to be associated with smooth muscle induced arterial dilation, as Ca2 + influx through TRPV4 activates ryanodine receptor, which in turn influences large conductance Ca2 + and voltage-activated potassium channels (BKCa) channels leading to vasodilation [24]. Members of the TRPM subunits are also represented in smooth muscle function, as TRPM3 agonist was shown to modulate contractile responses in the aorta [25]. In addition, TRPM4 is known to mediate pressure-induced vasoconstriction of the cerebral arterial myocytes, a process regulated by Ca2 + release from the IP3 receptor on the sarcoplasmic reticulum [26]. Myogenic tone is an intrinsic vasomotor mechanism through which resistance arteries respond to changes in intravascular pressure. Stretch-activated channels respond to changes in intravascular pressure by facilitating Ca2 + entry and/or by membrane depolarization, activating voltage-operated Ca2 + channels. Elevation of intracellular Ca2 + leads eventually to the constriction of the vessel, by activation of myosin light chain kinase and/or by protein kinase C (PKC)/Rho-kinase dependent mechanisms [12,27]. Among the 27 human TRP subunits, at least 11 (TRPC1, TRPC5, TRPC6, TRPV1, TRPV2, TRPV4, TRPM3, TRPM4, TRPM7, TRPP2, and TRPA1) are known to be mechanosensitive stretch-activated channels [12]. Recent studies in aged hypertensive mice suggest that TRPC channel suppression mitigates the myogenic constriction of the middle cerebral artery and the loss of the TRPC channel-dependent component of myogenic tone resulting in a diminished response to intraluminal pressure [28]. Furthermore, in rat cerebral arteries, smooth muscle depolarization and constriction response to increased intraluminal pressure was attenuated by down-regulation of TRPC6 [29]. The expression of TRPM4-like channels in cerebral artery myocytes can contribute to the depolarization and vasoconstriction of cerebral arteries, after activation by membrane-stretch [30]. Suppression of TRPM4 attenuated pressure-induced smooth muscle depolarization and myogenic vasoconstriction in intact rat cerebral arteries [31]. TRPM4 inhibition also resulted in decreased myogenic constriction and impaired autoregulation of cerebral blood flow in rats [32]. The role of PKC-dependent regulation of TRPM4 in the development of myogenic tone was demonstrated when suppression of TRPM4 resulted in the weakening of PKC-induced depolarization and vasoconstriction in cerebral arteries [33]. TRPV2 expression has been demonstrated in the aortic, mesenteric, and basilar arterial smooth muscle cells, where their down-regulation attenuated swelling-induced inward current and Ca2 + release [34]; however, there is no evidence of their role in the cerebral circulation. Endothelial cells form a semipermeable barrier allowing selective passage of molecules between the lumen and the interstitium. Increased endothelial permeability during inflammatory processes has been shown to be induced by actin polymerization-dependent endothelial cell rounding and by the formation of interendothelial gaps [25,35,36]. In addition, protein kinase C isoform (PKC) alpha is shown to be activated by Ca2 + influx into the cell, inducing endothelial contraction and disassembly of VE-cadherin junctions, leading to endothelial permeability changes [25,35,36]. TRPC1 and TRPC4 were among the first TRP subunits connected to abnormal permeability of endothelial cells, as TRPC4 knockout mice exhibited attenuated lung microvascular permeability in response to thrombin receptor activation, and TRPC1/C4 induced Ca2 + entry were proposed to disrupt the endothelial cell barrier and increase permeability in response to an inflammatory agonist [25,35,37]. Furthermore, TRPC6 was shown to activate Rho kinase through a PKC alpha mediated pathway, consequently leading to endothelial permeability [38]. Interestingly, vascular endothelial growth factor (VEGF) induced hyperpermeability of the endothelial barrier, which is a common denominator for many neurovascular diseases; was demonstrated to be mediated through the PLC-IP3 pathway, activating Ca2 + entry via TRPC1 [39]. Furthermore, it has been observed that Ca2 + entry through TRPM4 leads to the formation of interendothelial gaps and to hyperpermeability in the capillary segment of the pulmonary artery [40]. Similarly, pulmonary microvessel permeability was also connected to TRPM2 (a nonselective cation channel mediating oxidant-induced Ca2 + entry) activation by neutrophilic oxidants, a process regulated by PKC alpha [41]. However, the role of these channels in cerebral microvascular permeability is yet to be demonstrated. Investigations on the role of endothelial permeability in the functionality of the blood-brain barrier (BBB) have demonstrated some interesting findings. Changes in Ca2 + dynamics are now believed to influence BBB permeability as bradykinin, an inflammatory peptide increased Ca2 + oscillations resulting in hyperpermeability of the BBB [42]. Interestingly, this effect was abolished by interfering with connexin channels (i.e., through a peptide blocker) and also by the knockdown of connexin 37/40 [42]. The role of TRP channels in BBB permeability is indeed intriguing and observations indicate that controlling Ca2 + dynamics through these channels may attenuate the BBB permeability alterations associated with many neurological disorders. Endothelial expression of 21 TRP channels has been documented, and at least five are associated with the endothelial-dependent vasodilation and blood flow delivery [43]. TRPC4 was the first TRP subunit thought to be involved in endothelium-dependent vasodilatation, as its knockout mice showed reduced agonist-induced Ca2 + entry and vasorelaxation [44]. TRPA1, a Ca2 + permeable nonselective cation channel, activated by electrophilic compounds such as allicin and allyl isothiocynate (AITC), was also associated with endothelium-mediated tone regulation [45]. AITC induced dilation of cerebral vessels in a concentration dependent manner, an effect that was selectively attenuated by the TRPA1 channel blocker HC-030031 [45]. Interestingly, AITC-induced vasodilation is insensitive to nitric oxide synthase inhibition but was blocked by the small and intermediate conductance Ca2 + activated potassium (K+) channel blockers, apamin and TRAM34 [45]. In agreement with these results, a recent study demonstrated the correlation between the AITC-induced increase in Ca2 + signals and consequent dilation of cerebral arteries in a concentration-dependent manner [46]. In addition, carvacrol (a compound present in oregano) was shown to trigger a concentration-dependent increase of intracellular Ca2 + in cerebral arterial endothelial cells and induced dilation of cerebral arteries, an effect inhibited by the presence of Ca2 +-activated K+ channel blockers in the lumen and by the presence of the inwardly rectifying K+ channel blocker BaCl2 [47]. Although TRPA1 and TRPV3 were both expressed in endothelial cells, carvacrol induced dilation was inhibited only by TRPV blocker ruthenium red, whereas it was unaffected by TRPA1 antagonist [47]. Angiogenesis is the formation of new vessels from preexisting vessels, a process normally dormant during adulthood with the exception of wound healing and physiological formation of vessels. Angiogenesis as a therapeutic paradigm is a “double edged sword.” Proangiogenic therapy aims to increase new vessel formation to increase blood flow to the damaged tissue, enhancing or encouraging recovery; whereas, antiangiogenic therapies target tumor vascularization, a process critical for tumor metastasis and thereby a fascinating target for cancer treatment. Tumor vascularization has been linked to many different Ca2 + channels, as Ca2 + signaling is quintessential for cellular processes such as gene transcription, cell proliferation, migration, and angiogenesis [48]. As TRP channels influence intracellular Ca2 + concentrations impacting crucial cytosolic and nuclear events, a growing body of evidence points toward their role in cancer incitation and progression [48]. Tumor tissue expansion induces drastic changes in the cellular environment and TRP channels with their ubiquitous expression, and multidimensional activation patterns may be involved in the regulation of different stages of cancer cell development [48–50]. Out of the TRP channel subfamilies, endothelial TRPC1, TRPC4, TRPC6, TRPV4, and TRPM7 have been implicated in angiogenesis [48,51]. The first evidence for the role of TRPs in vessel formation came from a study demonstrating disrupted formation of intersegmental vessels during zebra fish development after TRPC1 knockdown [52]. TRPC1 was also shown to be a downstream component of the VEGF-induced angiogenesis pathway where it participated in the phosphorylation of extracellular signal regulated kinase (ERK) [52]. In addition, TRPC1 (along with TRPC4) was shown to be involved in tube formation by primary human umbilical vein endothelial cells (HUVECs) [53] and was also shown to regulate the growth of endothelial progenitor cells by participating in store-operated Ca2 + entry [54]. In the kidney, it has been demonstrated that TRPC4 loss in renal cell carcinoma (RCC) leads to impaired Ca2 + intake and diminished secretion of antiangiogenic protein thrombopsondin-1, enabling RCC progression [55]. Another study showed the role of TRPC4 in the transition of endothelial cells from a proliferating to quiescent phenotype by functioning as a stimulated Ca2 + entry channel [56]. The overexpression of TRPC6 increased proliferation and migration of human microvascular endothelial cells [57], whereas its inhibition arrested VEGF-induced proliferation and tube formation in HUVECs [58], delineating its role in VEGF-mediated angiogenesis. In addition, TRPC6 was found to cause a sustained elevation of intercellular Ca2 + in glioblastoma multiforme (GBM), which is coupled with the activation of calcineurin-nuclear factor of activated T-cell pathway [59]. Also, TRPC6 was elevated in clinical specimens of GBM, and knockdown of TRPC6 was related to reduced glioma growth, invasion, and angiogenesis [59]. Phosphatase and tensin homologue was shown to serve as a scaffold for TRPC6, enabling its cell surface expression and facilitating Ca2 + entry, inducing an increase in endothelial permeability and angiogenesis [60]. Stimulation by cyclic mechanical strain of pulsatile blood flow has been shown to activate the mechanosensitive TRPV4, promoting cytoskeletal remodeling and cell reorientation, processes critical for angiogenesis [61]. Activation of TRPV4 by fluid shear stress also lead to proliferation of vascular cells, and treatment with a specific TRPV4 activator, 4alpha-Phorbol 12, 13-didecanoate (4alphaPDD), triggered collateral growth and facilitated recovery in rats after femoral artery ligature [62]. Endothelial proliferation and angiogenesis are also influenced by magnesium dynamics, hence the magnesium transporter TRPM7 has also been linked to these processes. An inhibitory role of TRPM7 in the angiogenic process was revealed when its knockdown resulted in the proliferation of human microvascular endothelial cells, an effect modulated at least in part by the ERK pathway [63]. Taken together, these investigations have delineated several roles of TRP channels in endothelial cell proliferation and angiogenesis that could be targeted for developing effective proangiogenic and antiangiogenic therapies. Hypertension is considered a primary risk factor of vascular diseases and has been implicated in atherosclerosis, stroke, heart failure, and renal complications [64,65]. Among the several factors that contribute to the development of hypertension, dysregulation of Ca2 + homeostasis and the consequent vascular dysfunction are most common. Advances in the TRP channel field have enabled us to understand that these cation channels, and variations in their expression and/or regulation, are responsible for the development of many disorders, including hypertension. The TRP channel subunits TRPC1, TRPC3, TRPC5, TRPC6, TRPV1, TRPV4, TRPV5, TRPM4, TRPM6, TRPM7, and TRPA1 have all been connected to the development and/or progression of hypertension. TRPC3, TRPC5, and TRPM6 were found to be reduced significantly in patients after hypertensive intracerebral hemorrhage in comparison with controls [66]. In addition, the authors also found a correlation between the expression of TRPC3 and hypoxia inducible factor 1a, indicating its association with hypoxic conditions in the human cerebral tissue [66]. In spontaneously hypertensive rats, the expression of TRPC1, C3, and C5 were significantly increased in the mesenteric arteries and was associated with an increase in norepinephrine-induced vasomotion in comparison with normotensive rats [67]. Chronic treatment with candesartan or telmisartan significantly reduced the expression of these TRPs and norepinephrine-induced vasomotion in these animals [67]. Activation of the TRPC3 channel leads to myocyte depolarization and Ca2 + entry through VDCC resulting in vasoconstriction [68]. VDCC Ca2 + entry is suggested to be crucial for the autoregulation of cerebral blood flow and hence could be an interesting target to address dysfunctional vascular contractility, as during hypertension [68]. In addition, Xi et al. have demonstrated a mechanism of IP3-induced vasoconstriction of cerebral arteries, which occurs independent of SR Ca2 + release and mediated by TRPC3 channels and IP3 receptor-dependent I(Cat) activation [69]. Monocytes of erythropoietin-treated, hemodialysis patients revealed an increased expression of TRPC5 mRNA accompanied by increased systolic blood pressure, indicating a role of TRPC5 in erythropoietin induced hypertension [70]. In HUVECs, TRPC6 and TRPV1 mRNA expression levels were significantly increased after exposure to a pulsatile atheroprone shear stress flow in comparison to an atheroprotective flow profile [71]. Furthermore, the authors also found an association between TRPC6 mRNA expression and tumor necrosis factor-α mRNA in the human vascular tissue, delineating its connection to the inflammatory processes in the vasculature [71]. Recently, the involvement of TRPC6 and 20-hydroxyeicosatetraenoic acid (HETE) in the autoregulation of cerebral blood flow was elucidated under conditions of hypertension, where the authors described the loss of autoregulation in aged, Ang II-induced hypertensive mice along with exacerbated disruption of the BBB, increased neuroinflammation, and impaired hippocampal-dependent cognitive function [72]. In addition, it has been shown that 20-HETE activation of TRPV1, in part, mediates pressure-induced myogenic constriction and underlines 20-HETE-induced elevations in blood pressure and coronary resistance [73]. The connection between TRPV1 and intraluminal pressure changes were first described by Scotland et al. as an elevation in intraluminal pressure generated 20-HETE, activating TRPV1 on C fiber nerve endings and resulting in a myogenic response [74]. TRPV1 activation by dietary capsaicin enhanced endothelium dependent relaxation in mice, an effect absent in TRPV1 knockout mice [75]. Furthermore, chronic stimulation of TRPV1-activated PKA, resulted in increased eNOS phosphorylation, improved vasorelaxation, and the lowering of blood pressure in genetically hypertensive rats [75]. Remarkably, long-term administration of capsaicin significantly delayed the onset of stroke and increased survival time in spontaneously hypertensive rats, an effect mediated through eNOS-dependent vasorelaxation and induced by TRPV1 activation [76]. Moreover, long-term activation of capsaicin increased ATP binding cassette transporter A1 and reduced low-density lipoprotein-related protein1 expression in the aorta of atherosclerotic mice (ApoE−/−), reducing lipid storage and atherosclerotic lesions in the aorta, an effect absent in ApoE(−/−)/TRPV1 (−/−) double knockout mice [77]. Rodent experiments have suggested activation of the sympathetic nervous system through the osmosensitive channel TRPV4 to be responsible for the robust increase in blood pressure after water ingestion, observed in human subjects with impaired baroreflex function [78]. Even though TRPV4 has been shown to be involved in pulmonary vasoreactivity [79] and hypoxia-induced pulmonary hypertension [80], and that nitric oxide synthase-inhibition induced hypertension is exacerbated in TRPV4 knockout mice [81], its role in the cerebral vasculature and its dysfunction, remains to be clearly elucidated. The inhibition of WKN4, a protein serine/threonine kinase that positively regulates the TRPV5-mediated Ca2 + transport process, is believed to be involved in the pathogenesis of familial hyperkalemic hypertension [82]. Moreover, TRPV5 knockout mice present phenotypic defects of renal disease such as hypercalciuria and impaired bone mineral density, exemplifying the role of this channel in renal physiology, hypertension, and genetic disorders of the kidney [82,83]. The role of TRPM subunits in hypertension is mainly represented by TRPM4, 6, and 7, where TRPM4 was first shown to be increasingly expressed in ventricular samples of spontaneously hypertensive rats in comparison with normotensive controls [84]. Interestingly, TRPM4 has been also connected with the electrophysiological perturbations associated with cardiac hypertrophy, of which hypertension is an important risk factor [85]. TRPM4 knockout mice present dysregulation of blood pressure toward hypertensive levels as TRPM4 regulates catecholamine release from chromaffin cells, the dysfunction of which leads to increased sympathetic tone and hypertension [86]. Mg2 + influences many cellular functions and variations in Mg2 + in the serum and tissue has been known to inversely affect blood pressure [87]. As TRPM6 and TRPM7 are associated with Mg2 + transport in the tissue, they are also involved in the development of vascular dysfunctions and hypertension. Whereas TRPM6 is expressed in epithelial cells, TRPM7 is globally expressed and is known as an important regulator of Mg2 +. Membrane-associated Ang II- and aldosterone-regulated TRPM7 are involved in the DNA and protein synthesis in both rodent and human VSMCs [88]. Importantly, the expression of TRPM7 was blunted in spontaneously hypertensive rats, which resulted in decreased Mg2 + in the VSMC [89]. Similarly, in low intracellular Mg2 +-exhibiting mice, the expression of TRPM7 was significantly elevated in the vasculature, whereas its downstream target, anti-inflammatory annexin-1, was reduced [90]. These animals also exhibited endothelial dysfunction, changes in vascular structure, and inflammation, revealing the role of TRPM7 signaling in the maintenance of vascular integrity and function [90]. A recent investigation has also revealed the role of TRPM7 in vascular remodeling, where its expression increased with the pressure overload-induced vascular wall thickening [91]. It was also associated with simultaneous accumulation of macrophages in the adventitia and decreased expression of annexin-1, an effect reversed by TRPM7 inhibitor 2-aminoethoxydiphenyl borate [91]. TRPA1 are a class of mechanosensitive Ca2 + permeable channels that are activated by irritant chemicals and pungent natural compounds [92,93]. L-type calcium channel antagonist 1,4-dihydropyridines, including nifedipine, nimodipine, nicardipine, and nitrendipin, commonly used in the treatment of hypertension, have been shown to exert powerful excitatory effects on TRPA1 channels [92]. In addition, nifedipine is known to induce large elevations of Ca2 + in mouse nociceptors expressing TRPA1, an effect abrogated in the TRPA1 knockout mice [92]. Ischemia refers to the inadequate supply of oxygen and nutrients to an organ, resulting in cell death and degeneration. Stroke is the fourth major cause of death and is the leading cause of adult disability in the Western population [94]. As stroke results from the blockage or disruption of a supplying blood vessel, vascular dysfunction is a vital risk factor in its development and during recovery. Hypoxia resulting from ischemia, activates a myriad of cellular responses including the rise in intercellular Ca2 + [95]. As the rise in intercellular Ca2 + depends on the activation of Ca2 + permeable channels and/or by the activation of intercellular Ca2 + stores, TRP channels, with their known influence on these mechanisms, are likely to play a key role. The first demonstration of the involvement of a TRP subunit in hypoxia was the observation that TRPM7 suppression blocked Ca2 + uptake, reactive oxygen species production and anoxic cell death in cortical neurons subjected to prolonged oxygen glucose deprivation [96]. Electrophysiological investigation on acute hippocampal slices showed that TRP channels are activated by cellular stress, contributing to ischemia-induced membrane depolarization, intracellular calcium accumulation, and cell swelling [97]. Likewise, TRPM2 mRNA expression was increased at 1 and 4 weeks following ischemic injury in a rat middle cerebral artery occlusion model of stroke [98]. In mouse hippocampal neurons, it was demonstrated that TRPM7 channels were involved in detecting the reduction in extracellular divalent ions, known to contribute to neuronal death after brain ischemia [99]. TRPM7 suppression by shRNA made neurons resistant to neuronal damage after brain ischemia, preserving neuronal morphology and function, leading to long-term potentiation and animal survival [100]. However, an assessment of 16 tag-single-nucleotide polymorphisms of the TRPM7 gene in humans showed no evidence for its role as a risk factor for ischemic stroke incidence [101]. An increase in TRPV4 expression in the cortical and hippocampal astrocytes of adult rat was shown to coincide with the development of astrogliosis after ischemia [102]. In addition, cultured hippocampal astrocytes have been found to respond to the TRPV4 activator 4-alpha-phorbol-12,-13-didecanoate as detected by an increase in intracellular Ca2 +; this effect was augmented after hypoxic/ischemic injury, demonstrating the possible role of TRPM4 in astroglial reactivity after ischemia [102]. An investigation on a primate model of stroke has revealed promising results that Tat-NR2B9c, a PSD-95 interfering peptide known to disrupt TRPM7/PSD-95/neuronal NOS pathway, reduced stroke volume, maintained gene transcription, and preserved neurological function after ischemia [103,104]. The emerging role of TRP channels in neuronal injury development after stroke opens up a potential paradigm for clinical interventions in patients, extending even beyond the acute phase of stroke. However, the expressional, regulatory and functional characteristics of the many different TRP subunits need to be thoroughly elucidated so that a feasible therapeutic strategy could be derived for interventions in stroke pathology. Our understanding of TRP channels has exploded in the past decade (> 1600 manuscripts annually [105]), fueled by its potential as a drug target. However, the conundrum surrounding TRP channel functionality and its connection to human disease is currently underdeveloped. The role of TRP channels in the cerebral circulation and in cerebrovascular diseases is still in its infancy. As TRP channels have been implicated in myogenic tone blood flow control along with the permeability of the BBB, collateral formation, angiogenesis and vascular remodeling, its significance in the cerebral circulation and cerebrovascular disorders have gained appreciation. The varied and global expression of TRP channels demonstrate its multifaceted roles in cellular physiology and at the same time presents itself as a challenging barrier for drug design and implementation. This task is further complicated by the ability of TRPs to heteromultimerize and to interact with other intercellular proteins (channelosomes). The fact that many of TRP members are activated by natural compounds (e.g., carvacrol, capsaicin) is enthralling, as plant extracts have been used in traditional medicine for many centuries. Hence, it is conceivable that the regulation of TRP channels and subsequent intervention of their associated pathologies may be possible through dietary supplements via naturally occurring compounds, thereby minimizing the risk of side effects in patients. Given the ubiquitous nature and the presence of multidimensional activation pathways, a comprehensive understanding of TRP channel biology remains the “sine qua non” for extracting its therapeutic potential.
Connecting TRP Channels and Cerebrovascular Diseases
2 Libin Cardiovascular Institute, University of Calgary, Calgary, Alberta, Canada
3 Department of Physiology and Pharmacology, University of Calgary, Calgary, Alberta, Canada
* Corresponding author: dwelsh@ucalgary.ca
Abstract
Overview: TRP Channels: Types, Distribution, and Function
TRP Channels in Diseases
TRP Channels in Smooth Muscle Cells
Role in Smooth Muscle Proliferation
Role in Smooth Muscle Control of Blood Flow
Role in Myogenic Tone
TRP Channels and Endothelial Function
TRP Channel and Vascular Barrier Function
TRP Channels and Vasodilatation
TRP Channels: Endothelial Proliferation, Angiogenesis, and Vascular Remodeling
TRP Channels and Hypertension
TRP Channels and Stroke
Future Directions
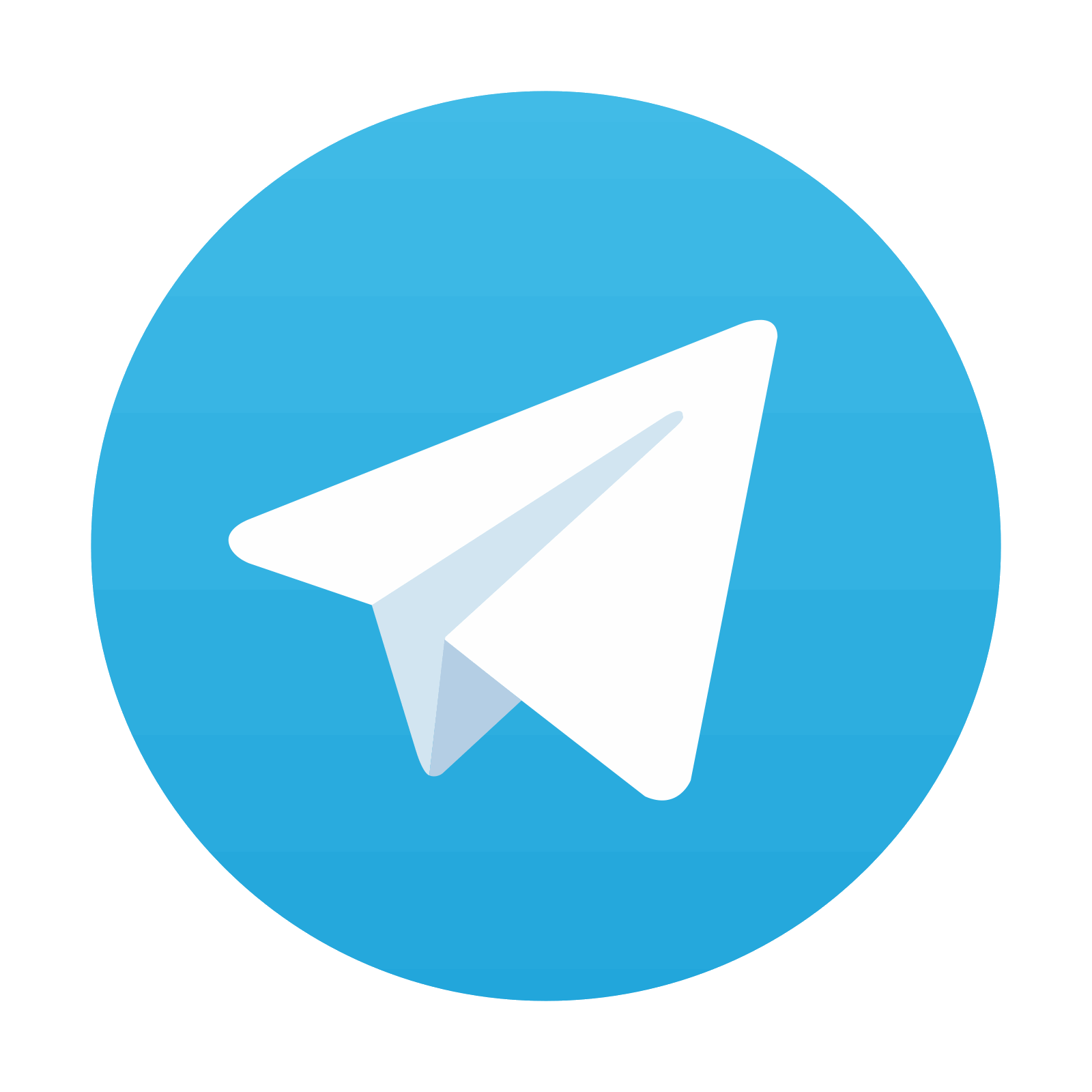
Stay updated, free articles. Join our Telegram channel
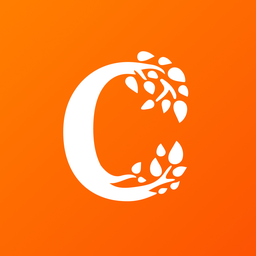
Full access? Get Clinical Tree
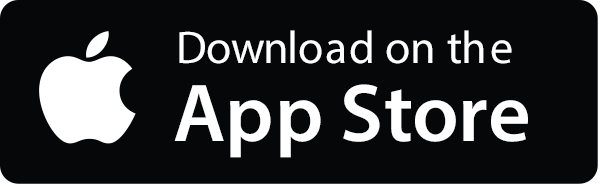
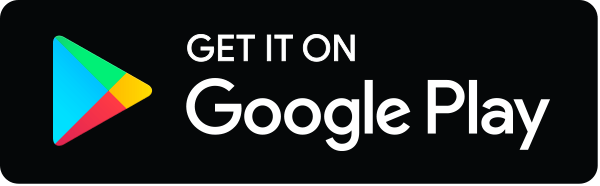