and Miguel A. R. B. Castanho2
(1)
Instituto de Bioquímica Médica Leopoldo de Meis, Federal University of Rio de Janeiro, Rio de Janeiro, Rio de Janeiro, Brazil
(2)
Institute of Biochemistry and Institute of Molecular Medicine, School of Medicine, University of Lisbon, Lisbon, Portugal
Catabolism is the group of metabolic pathway s in which the chemical energy contained in the nutrient molecules is transferred to other compounds, especially ATP , which in turn provides the energy for different cellular processes such as the synthesis of new biomolecules, ion transport across cellular membranes , and muscle contraction .
In Sect. 6.2, we discussed in detail the mechanism of ATP synthesis through oxidative phosphorylation , starting with the electron transfer from the reduced coenzymes NADH and FADH2 going to the components of the respiratory system. In this chapter we will present the metabolic pathway s along which the oxidation of the nutrient molecules results in the reduction of NAD+ and FAD , generating the reduced coenzymes that feed the electron transport system.
7.1 An Overview of Catabolism
Carbohydrates, lipids , and proteins are the major constituents of food and serve as fuel molecules for the human body. The net energy yielded by the metabolism of each of these nutrients was determined for humans in the end of the nineteenth century, by the pioneer work of Wilbur O. Atwater, who developed a human respiration calorimeter (see Box 7.1) and obtained the values of 4.0, 4.0, and 8.9 kcal/g for carbohydrate, protein , and fat, respectively, values that are still considered valid today.
Box 7.1: Wilbur O. Atwater and the Studies on Human Nutrition
Atwater started his scientific career in agricultural chemistry, studying fertilizers, but his major contributions for the advancement of science came when he changed his scientific interest to human nutrition after he spent a period in Carl von Voit’s laboratory, in Germany. Atwater developed systematic studies on the composition and the digestibility of foods as well as on the kind of foods people from different ethnic groups in different areas were eating. At that time, part of his interest was focused on finding out how poor people could make more economical choices of food maintaining its nutritional value. In 1893, Atwater’s group developed a respiration calorimeter for studies of human metabolism , known as the Atwater–Rosa–Benedict’s human calorimeter (see figure). This system allowed them to measure the metabolizable energy contributed by each of the nutrient molecules.
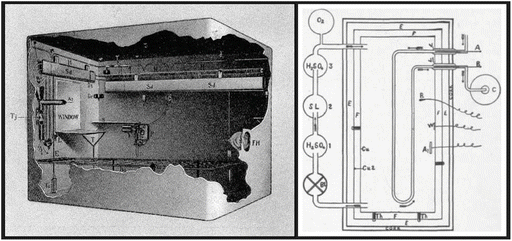
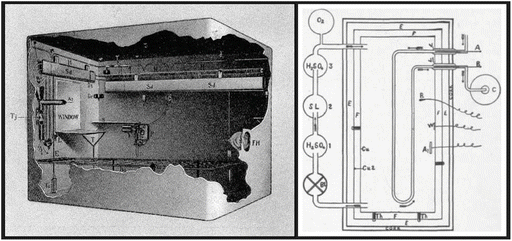
Inside view of the Atwater–Rosa–Benedict’s human respiration calorimeter (image from http://vlp.mpiwg-berlin.mpg.de/references?id=lit15620&page=p0213) and the diagrammatic representation of its components (reproduced from the book The Elements of the Science of Human Nutrition, by Lusk G, 1917). The studied subject entered a copper chamber with a capacity of 1123 l and lay on a bed, and the chamber was hermetically closed. The air within the chamber passes through three bottles previously weighed containing (1) sulfuric acid, which removes the water; (2) moist soda lime, which removes the CO2 ; (3) and sulfuric acid again, which absorbs the moisture taken from the soda lime. The gain in weight of bottle 1 represents water absorbed, and the gain in weight of bottles 2 and 3 equals the CO2 absorbed. O2 was automatically fed into the system by cylinder, and O2 consumption was calculated by weighting the cylinder before and at the end of the experiment
The main products of carbohydrates, lipids , and protein digestion (monosaccharides, fatty acid s , and amino acid s , respectively) reach the bloodstream and enter the cells, where they are firstly metabolized through a specific catabolic route that converges to a central pathway in the metabolism , the tricarboxylic acid (TCA) cycle (Fig. 7.1), also referred to as the Krebs cycle (see Sect. 7.2.3).
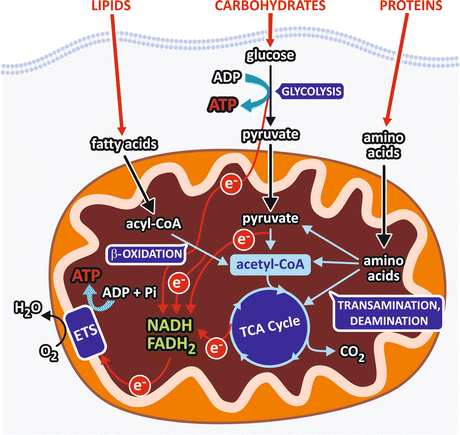
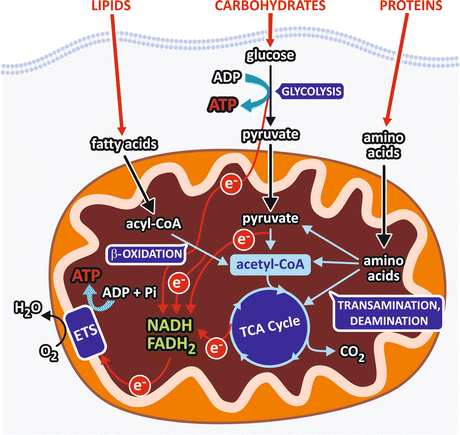
Fig. 7.1
Schematic representation of a generic cell showing the catabolic pathways. Carbohydrate digestion generates monosaccharides, mainly glucose , which is degraded through glycolysis into two pyruvate molecules. In this pathway, an oxidative step is coupled to NAD+ reduction, generating NADH that feeds the respiratory chain (electron transport system, ETS) with electrons (e−) that ultimately reduce O2 to H2O. Pyruvate is converted to acetyl-CoA in the mitochondrial matrix in a reaction involving an oxidative decarboxylation with concomitant NAD+ reduction to NADH. Degradation of fatty acid s occurs through the pathway known as β-oxidation , which sequentially removes from the fatty acid chain a two-carbon fragment, in the form of acetyl-CoA, with concomitant reduction of FAD and NAD+. Amino acids, the products of protein hydrolysis, undergo transamination or deamination reactions that remove their amino group and generate intermediates of the central metabolic pathway . The circles in the electron representation are used to indicate that they are not free in the cellular medium but are transferred by the compounds of each pathway
The formation of a molecule of acetyl-coenzyme A (acetyl-CoA ) is the convergence point of the degradation pathways of monosaccharides, fatty acid s , and some of the amino acid s (Fig. 7.1). Then, acetyl-CoA is completely oxidized to CO2 in the TCA cycle, to which the products of the degradation of other amino acid also converge. In the oxidative reactions of the TCA cycle, electrons are transferred from the TCA cycle intermediates to NAD+ or FAD , generating NADH and FADH2 that in turn will transfer the electrons to O2 through the respiratory system (see Sect. 6.2.2). Thus, in the overall process, O2 is consumed and CO2 is produced. This process is called cellular respiration , in analogy to the physiological term respiration, which is attributed to the gas exchanges that occur in the lungs.
The specific pathways of fatty acid s and glucose degradation, the β-oxidation and glycolysis , respectively, also have oxidative steps in which FAD and/or NAD+ are reduced (Fig. 7.1). On the other hand, the reactions of amino acid metabolism that generates the intermediates of the central metabolic pathway consist basically in transamination or deamination reactions that remove the amino group from the amino acid molecules, followed in some cases by carbon skeleton rearrangements.
In this chapter we will first focus on the central pathway of acetyl-CoA oxidation, the TCA cycle, to which all the nutrient degradation products ultimately converge to feed the electron transport system. Then, we will describe each specific pathway for the degradation of monosaccharides, fatty acid s , and amino acid s .
It is important to bear in mind that the use of each nutrient molecule as a source of energy for ATP synthesis depends on the metabolic status (Fig. 7.2). For example, after a balanced meal, the increase in blood glucose concentration triggers a series of regulatory events that select the carbohydrates as the main class of nutrients to be used as energy source, while fats are stored in the adipose tissue (see Chap. 8). In contrast, during the intervals between the meals or during fasting or low-carbohydrate diets, fatty acid s are mobilized from the adipose tissue and become the major source of energy for most of the tissues in the body (see Chap. 9).
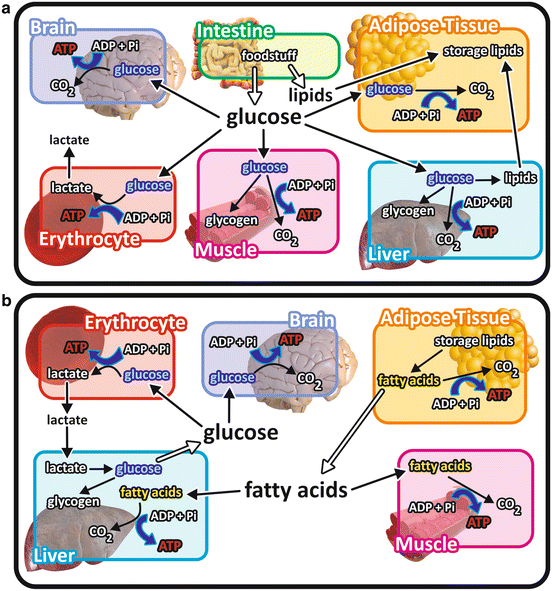
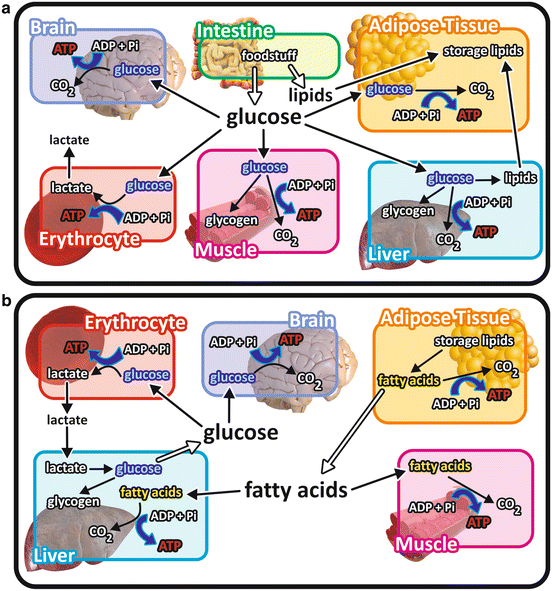
Fig. 7.2
Overview of the integration of metabolism in different metabolic situations emphasizing the catabolism of the different nutrient molecules. (a) After a balanced meal, carbohydrate catabolism is the main pathway for ATP synthesis in virtually all the cells in the body. Ingested lipids are stored in the adipose tissue. The excess of glucose is stored as glycogen in muscle and liver cells or converted into lipids in the liver and in the adipose tissue. (b) When glucose concentration decreases in the blood, fatty acid s are mobilized from the adipose tissue, becoming the main energy source for most of the tissues. Cells lacking mitochondria , as erythrocytes , or isolated from systemic circulation, as those of the central nervous system , are exceptions and maintain glucose catabolism in this situation
7.2 Tricarboxylic Acid Cycle: The Central Pathway for the Oxidation of the Three Classes of Nutrient Molecules
In aerobic metabolism , the products of nutrients’ degradation converge to the TCA cycle, a central pathway consisting in eight reactions (Fig. 7.3). In this pathway, the acetyl group of acetyl-CoA resulting from the catabolism of monosaccharides, fatty acid s , or amino acid s , is completely oxidized to CO2 with concomitant reduction of the electron-transporting coenzymes (NADH and FADH2 ).
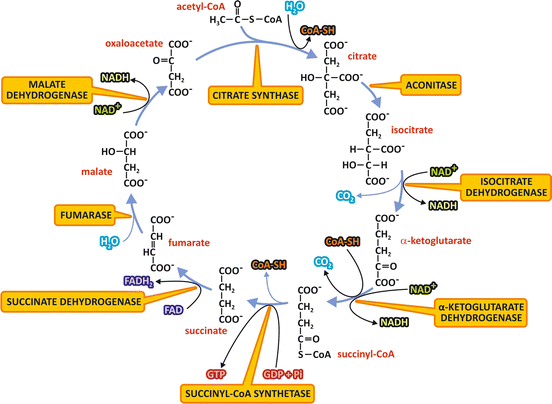
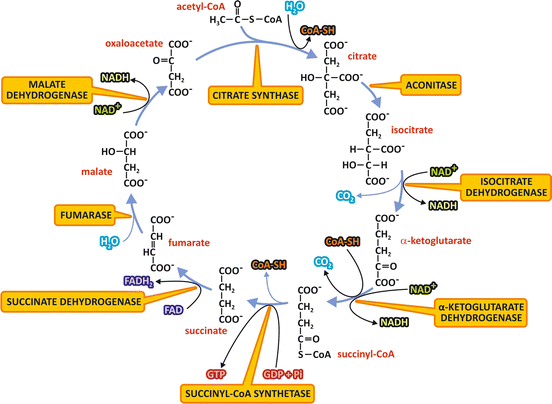
Fig. 7.3
Reactions of the TCA cycle. The NAD+ or FAD molecules reduced to NADH or FADH2 are indicated at the respective oxidation reactions, as well as the CO2 released at the decarboxylation reactions. The GTP molecule synthesized by substrate-level phosphorylation is also shown. The names of the enzymes are highlighted in yellow boxes. Remind that succinate dehydrogenase is the Complex II of the electron transport system
7.2.1 TCA Cycle Reactions
We start the description of TCA cycle reactions with the condensation of acetyl-CoA and oxaloacetate , which generates citrate (Fig. 7.3). The next seven steps, which regenerate oxaloacetate, include four oxidation reactions in which NAD+ or FAD are reduced to NADH or FADH2 , whose electrons will be then transferred to O2 in the respiratory chain . Additionally, a GTP or an ATP molecule is directly formed by the mechanism of substrate-level phosphorylation (see Sect. 6.1).
The condensation of the two-carbon acetyl group of acetyl-CoA with the four-carbon molecule oxaloacetate , forming citrate , a six-carbon molecule, is catalyzed by the enzyme citrate synthase . A thioester intermediate, citroyl-CoA, is formed in the active site of the enzyme, and its hydrolysis makes the reaction highly exergonic. The coenzyme A released in this reaction may be recycled in the conversion of pyruvate to acetyl-CoA by pyruvate dehydrogenase complex (see Sect. 7.3).
Citrate is then transformed to isocitrate, in a reaction catalyzed by the enzyme aconitase.
The next reaction is an oxidative decarboxylation catalyzed by isocitrate dehydrogenase, which converts the six-carbon compound isocitrate in the five-carbon molecule α-ketoglutarate , with the release of CO2 and reduction of an NAD+ molecule.
The next step is also an oxidative decarboxylation, the conversion of α-ketoglutarate in the four-carbon molecule succinyl-CoA , catalyzed by the enzyme complex α-ketoglutarate dehydrogenase. In this reaction, CO2 is released, and NAD+ works as the electron acceptor, with the subsequent binding of coenzyme A through a thioester bond forming succinyl-CoA. It is interesting to notice that α-ketoglutarate dehydrogenase is structurally similar to the pyruvate dehydrogenase complex, the enzyme complex that catalyzes the conversion of pyruvate into acetyl-CoA (see Sect. 7.3.1).
Succinyl-CoA is then converted to succinate by succinyl-CoA synthetase. In this reaction, the hydrolysis of the thioester bond of succinyl-CoA with concomitant enzyme phosphorylation is coupled to the transfer of a phosphate group bound to the enzyme to GDP or ADP through the mechanism of substrate-level phosphorylation.
Succinate dehydrogenase oxidizes succinate to fumarate . This enzyme is the Complex II of the respiratory system (see Sect. 6.2), a FAD -linked enzyme through which the electrons from succinate enter in the respiratory chain .
Then, fumarate is hydrated to form malate by the enzyme fumarase.
Finally, oxaloacetate is regenerated by the oxidation of malate catalyzed by malate dehydrogenase, with concomitant reduction of NAD+ .
It is important to point out that although the O2 does not participate directly in the TCA cycle, the cycle only operates in aerobic conditions since the oxidized NAD+ and FAD are regenerated in the respiratory chain .
7.2.2 TCA Cycle as a Dynamic Pathway
The TCA cycle is not a pathway that is only fed by acetyl-CoA . Likewise, CO2 is not its unique end product. Different four- and five-carbon metabolic intermediates may enter the cycle at different points to be oxidized, as occurs, for instance, with the amino acid glutamate after being converted to α-ketoglutarate by a transamination reaction (for details, see Sect. 7.5). The same occurs with the other amino acids , which are also converted in TCA cycle intermediates.
Besides receiving metabolic intermediates to be oxidized, the TCA cycle has also an anabolic role in metabolism , since many of its intermediates may be withdrawn from the cycle to be used as the precursors of biosynthetic processes (see Box 7.2). Due to its role in both catabolism and anabolism, the TCA cycle is considered an amphibolic pathway.
Box 7.2: The Anabolic Role of the TCA Cycle
Many TCA cycle intermediates may be used as precursors for biosynthetic pathways (see figure). Citrate leaves mitochondria yielding acetyl-CoA for the synthesis of fatty acid s and other lipids (see Sect. 8.3). α-ketoglutarate and oxaloacetate are the precursors of several amino acid s . The synthesis of porphyrins starts with succinyl-CoA. Oxaloacetate generates glucose through the pathway of gluconeogenesis (see Sect. 9.3.1) and is also the precursor of purines and pyrimidines that form the nucleotides . It is important to mention that when the TCA cycle intermediates are withdrawn for biosynthetic processes, a replacement mechanism should operate to avoid impairment of the oxidative metabolism . This occurs through replenishing reactions, known as anaplerotic reaction s , in which pyruvate or phosphoenolpyruvate (PEP ) are carboxylated, generating oxaloacetate or malate (see figure).
The most important of these reactions is the one catalyzed by pyruvate carboxylase (see Sect. 9.3.1). This reaction is highly regulated by acetyl-CoA , so that when this molecule accumulates, indicating that cycle intermediates are not sufficient to perform its complete oxidation, pyruvate is shifted toward the formation of oxaloacetate , increasing the capacity of the TCA cycle to oxidize acetyl-CoA. Pyruvate may also be converted to malate by the malic enzyme . The other anaplerotic reaction is that catalyzed by PEP carboxykinase, which generates oxaloacetate from PEP with the transfer of the phosphate from PEP to GDP , forming also a GTP molecule. This reaction occurs in the opposite direction in the gluconeogenesis pathway (see Sect. 9.3.1).
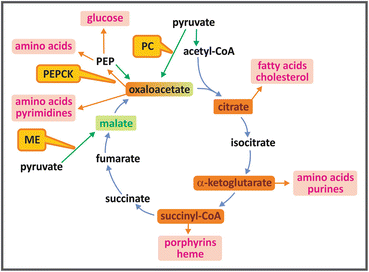
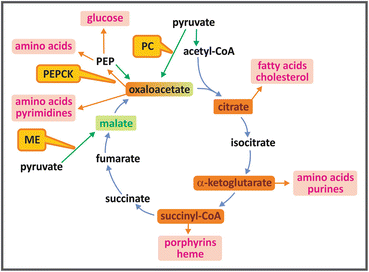
TCA cycle intermediates shown in orange boxes may be used as precursors for the synthesis of fatty acid s , cholesterol and steroids, amino acid s , purines and pyrimidines, porphyrins, and heme. Anaplerotic reactions (shown by green arrows), catalyzed by the enzymes pyruvate carboxylase (PC), phosphoenolpyruvate carboxykinase (PEPCK ), or malic enzyme (ME), replenish the cycle, maintaining its oxidative capacity
7.2.3 A Historical Overview of the TCA Cycle Discovery
The TCA cycle is also known as the “Krebs cycle” in recognition of the contribution of Sir Hans Krebs to its discovery (see Box 7.3). As stated in Krebs obituary, written by J. R. Quayle, “his life’s work, published in over 360 publications, spanned a whole era of biochemistry, namely, the elucidation of the major part of central intermediary metabolism and its regulation … Perhaps his greatest contribution to biological sciences as a whole was his discovery of the concept of metabolic cycles, how to recognize them, how to establish them unequivocally, and how to delineate and to assess their physiological function.”
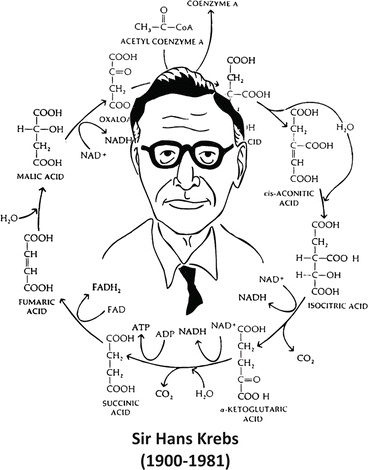
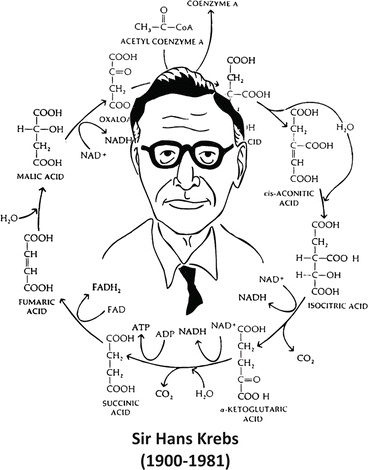
Box 7.3: Sir Hans Krebs and the Discovery of the TCA Cycle
Sir Hans Krebs was awarded the Nobel Prize in Physiology or Medicine in 1953, for the discovery of the TCA cycle. The prize was shared with Fritz Lipmann, who discovered the coenzyme A and its importance for the intermediary metabolism (see Box 7.4). Krebs began the studies that led him to the discovery of the TCA cycle working with Otto Warburg (see Box 6.4), who introduced to him the technique of using tissue slices for metabolic studies. This experimental procedure allowed working with intact cells in controlled media with free gas exchange between cells and the medium, an approach only possible before for microbiological studies. Using this technique, Krebs could propose for the first time a cycled pathway in the intermediate metabolism, the urea cycle (see Sect. 7.5). In the case of the TCA cycle, Krebs based his proposal on several observations carried out during the decade of 1930. In 1935, Albert Szent-Gyorgyi discovered the sequence of reactions from succinate to fumarate to malate to oxaloacetate (see figure), showing that these dicarboxylic acids present in animal tissues stimulate the O2 consumption. Two years later, Carl Martius and Franz Knoop found the sequence from citrate to α-ketoglutarate to succinate. Krebs integrated these findings adding his own observations about the effect of the addition of tricarboxylic acids to slices of pigeon breast muscle . He found that these acids even in very low concentrations promoted the oxidation of a much higher amount of pyruvate in the muscle slices, suggesting a catalytic effect of these compounds. He also observed that malonate, an inhibitor of succinate dehydrogenase, inhibited the oxidation of pyruvate and that the addition of fumarate to the medium in this condition restored pyruvate consumption (see figure). Assembling these information, Krebs elegantly showed the cyclic nature of the pathway.
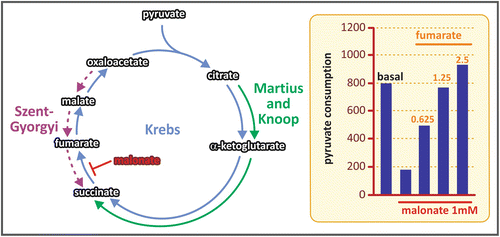
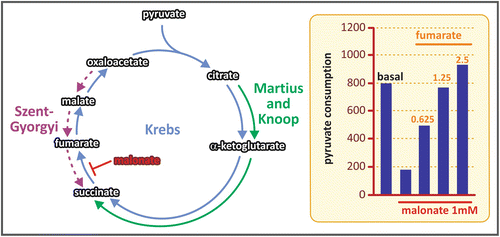
Sequence of reactions described by Szent-Gyorgyi (purple), Martius and Knoop (green), and Krebs (blue). The graph in the right shows the results of the crucial experiment done by Krebs that proved the cycled nature of the reactions for pyruvate oxidation: the inhibition of pyruvate consumption by the addition of malonate (an inhibitor of the enzyme succinate dehydrogenase) was abolished by fumarate addition to the system
When Krebs proposed the TCA cycle, in 1937, he thought that citrate was synthesized from oxaloacetate and pyruvate (see Box 7.3). Thus, the TCA cycle was seen as a pathway that serves only for carbohydrate oxidation, since pyruvate was known to be the product of carbohydrate metabolization (see Chap. 6). The importance of the TCA cycle in the oxidation of other compounds, such as the fatty acid s , was only realized after three important discoveries in the middle of the twentieth century: (a) the isolation of coenzyme A (CoA ), by Fritz Lipmann, in 1945 (see Box 7.4); (b) the isolation of acetyl-CoA by Feodor Lynen, in 1951; and (c) the subsequent work by Joseph Stern, Severo Ochoa, and Lynen, showing that the reaction between acetyl-CoA and oxaloacetate forming citrate was accompanied by the generation of stoichiometric amounts of sulfhydryl groups, indicating that acetyl-CoA was the molecule that donated the acetyl group to oxaloacetate.
Since acetyl-CoA is the product of the oxidation of pyruvate (and carbohydrates) (see Sect. 7.3) and fatty acid s (see Sect. 7.4), as well as being it generated in the metabolization of some amino acid s (see Sect. 7.5), one can recognize acetyl-CoA as the convergence point of the oxidative metabolism (see Fig. 7.1).
Box 7.4: Fritz Lipmann and the Discovery of Coenzyme A
The discovery of coenzyme A led Fritz Lipmann to be awarded the Nobel Prize in Physiology or Medicine in 1953. Lipmann dedicated many years of his scientific career working on the process of ATP synthesis . In 1941, he published a landmark paper in which he introduced the term “energy-rich phosphate bond” and established the basis for the understanding of the substrate-level phosphorylation mechanism of ATP synthesis (see Chap. 6). As a follow-up to these studies, Lipmann got interested in the capacity of some molecules to transfer acetyl groups, which he thought would be an important step in some biosynthetic processes. In the search for this “activated acetate,” Lipmann found a dialyzable, heat-stable factor present in all tissues he studied, which could not be replaced by any other known cofactor.
He purified and identified this new coenzyme and named it coenzyme A , in which “A” is related to the activation of acetate. Coenzyme A is composed of a phosphoadenosine diphosphate linked through a phosphoester bond to a pantothenic acid, which is in turn bound through an amide linkage to a β-mercaptoethylamine (see figure).
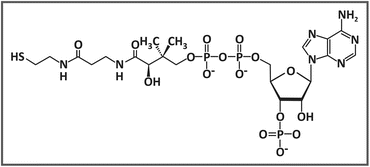
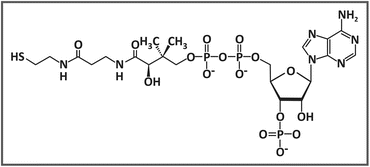
7.2.4 Regulation of the TCA Cycle
The rate of the TCA cycle is basically regulated by the flux of the dehydrogenases —isocitrate dehydrogenase, α-ketoglutarate dehydrogenase, succinate dehydrogenase, and malate dehydrogenase. The activities of the dehydrogenases, in turn, are determined by the mitochondrial ratios NADH /NAD+ and FADH2 /FAD , which depend on the ATP /ADP ratio. Therefore, when the concentration of ATP increases, the electron flow through the electron transport chain slows and the NADH/NAD+ and FADH2/FAD ratios increase, leading to a decrease in TCA cycle rate. On the other hand, when ATP is used, the increase in ADP levels allows the electron transport, increasing NADH and FADH2 oxidation and then enhancing TCA cycle rate.
7.3 Catabolism of Carbohydrates
Human diet contains different carbohydrates, including polysaccharides , such as starch and glycogen ; disaccharides, such as sucrose, lactose, and maltose ; and monosaccharides, such as glucose and fructose . Polysaccharides and disaccharides are broken in the digestive tract to their respective monosaccharides, which together with the free monosaccharides present in the diet reach the bloodstream and enter the cells. Inside the cells, monosaccharides are converted to a phosphorylated derivative that is degraded in two pyruvate molecules through a metabolic pathway named glycolysis (Fig. 7.4).
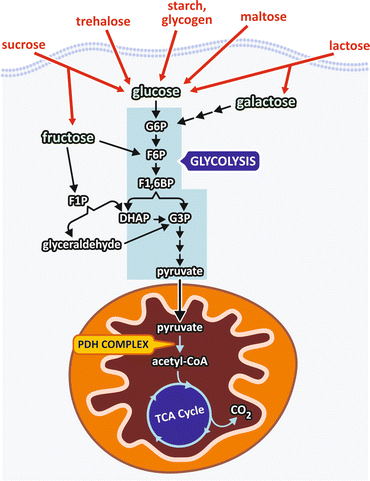
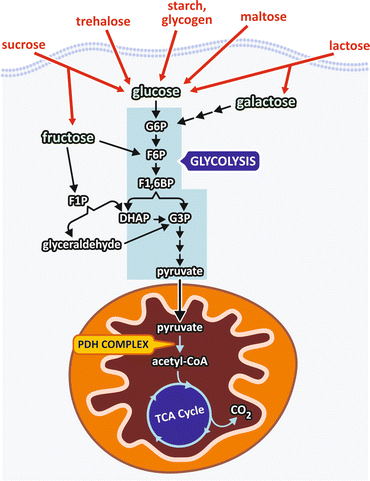
Fig. 7.4
Dietary carbohydrates are digested and reach the bloodstream mainly as monosaccharides that enter the cells where they are phosphorylated and degraded to pyruvate through the pathway named glycolysis . Pyruvate is transported into the mitochondria where it is converted to acetyl-CoA , which in turn is completely oxidized in the TCA cycle. G6P, glucose -6-phosphate ; F6P, fructose -6-phosphate; F1,6BP, fructose-1,6-bisphosphate ; F1P, fructose-1-phosphate; G3P, glyceraldehyde -3-phosphate; DHAP dihydroxyacetone phosphate
It is important to mention that besides being the pathway for carbohydrate degradation in aerobiosis, glycolysis also affords ATP synthesis in the absence of oxygen (see Chap. 6).
7.3.1 Carbohydrate Oxidation Reactions
Glycolysis itself is composed of ten reactions, which are presented in detail in Sect. 6.1.3. Thus, in this section we will focus our attention in the conversion of pyruvate to acetyl-CoA , the connecting point between glycolysis and the TCA cycle.
Pyruvate molecules, generated in the cytosol as the product of either glycolysis or the degradation of some amino acid s , need to be transported to the mitochondrial matrix where it is converted to acetyl-CoA (Fig. 7.4).
The oxidative decarboxylation of pyruvate to form acetyl-CoA is catalyzed by one of the largest cellular multienzyme complexes known, the pyruvate dehydrogenase complex (PDH ), with about 50 nm in diameter and a molecular mass of almost 10 MDa (Fig. 7.5a). Eukaryotic PDH is composed of multiple copies of four distinct proteins , three of them presenting enzymatic activity, E1 (pyruvate decarboxylase), E2 (dihydrolipoyl acetyltransferase), and E3 (dihydrolipoyl dehydrogenase) and the fourth, E3-binding protein (E3BP), which mediates E3 integration in the complex. Additionally, regulatory kinases and phosphatases are also associated to PDH complex.
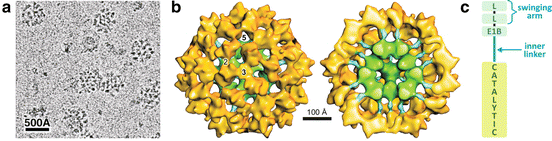
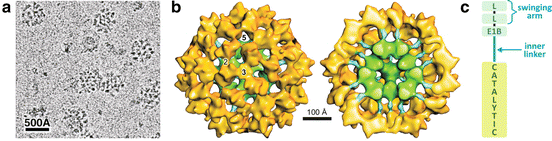
Fig. 7.5
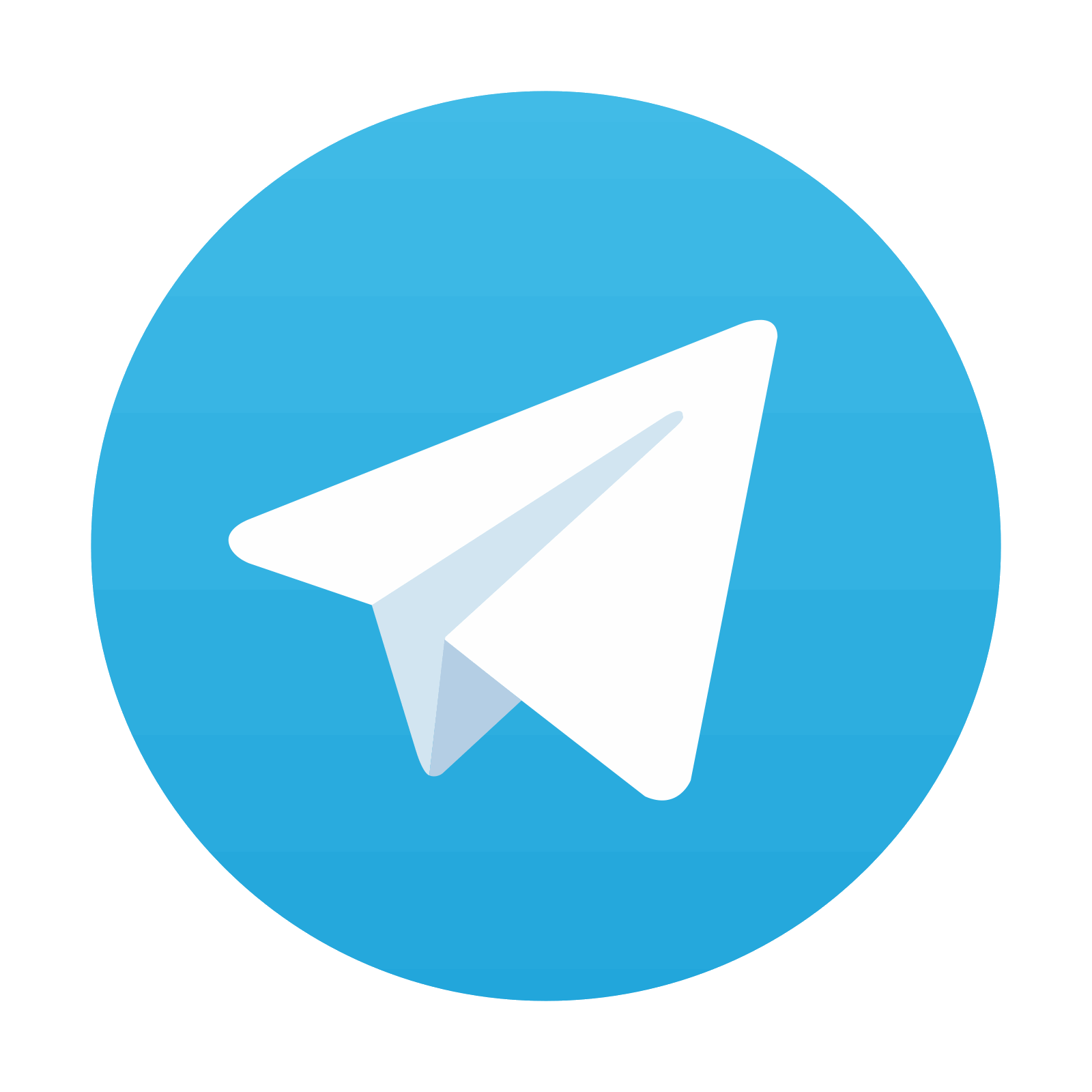
(a) Cryoelectron microscopy of bovine PDH showing the multienzyme complex with ~ 500 Å. (b) Reconstructed structure of the reconstituted complex and its half section (right) showing the dodecahedral core formed by E2 catalytic domains (green) and E2 inner linker (blue) that connects the core to the E1–E3 outer shell (yellow). (c) Diagrammatic representation of E2 four structural domains connected by linkers: the catalytic domain, the E1-binding domain (E1B), and the two lypoil domains (L) that form the “swinging arm.” (a) and (b): reproduced with permission from Zhou et al. Proc. Natl. Acad. Sci. USA 98:14802–14807, 2001
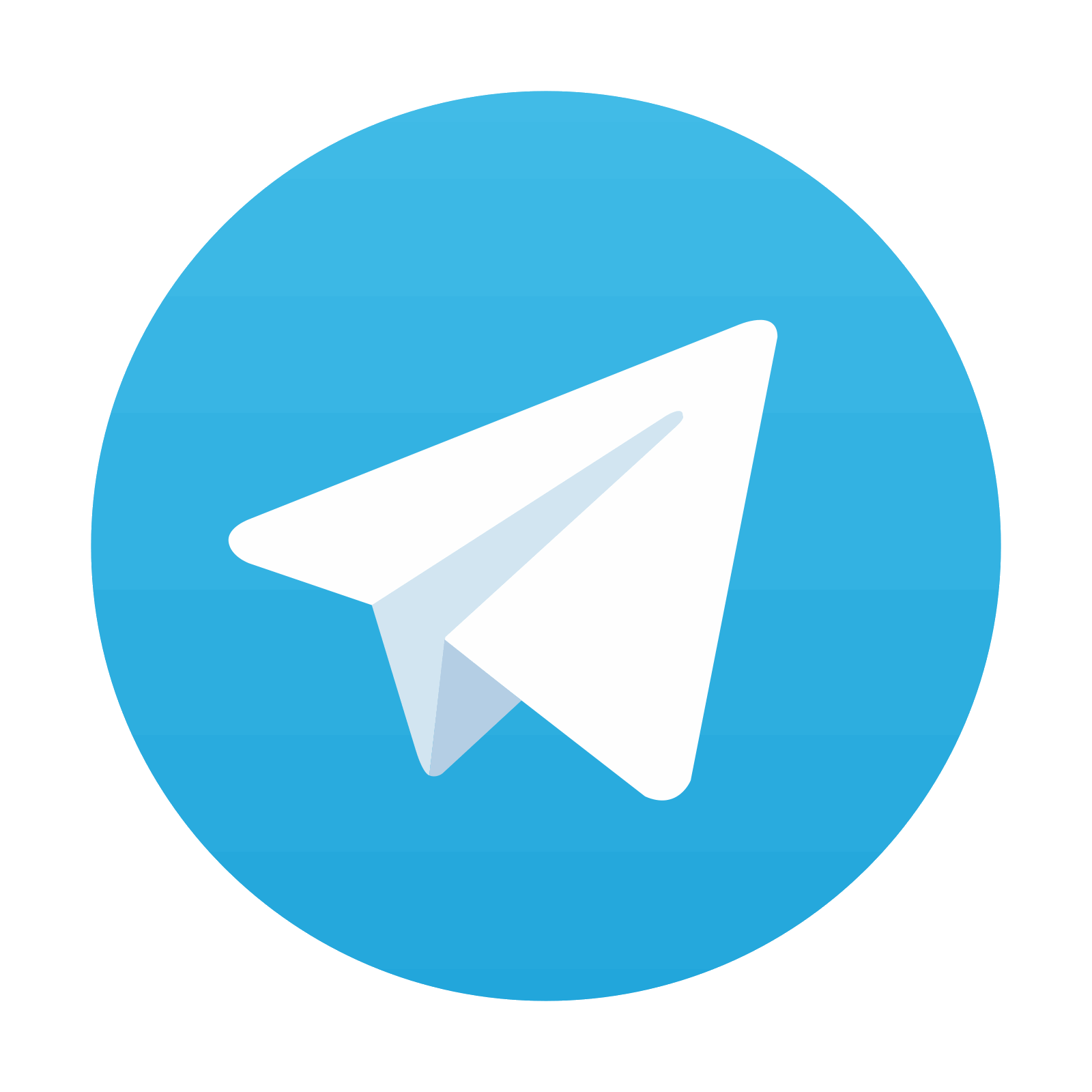
Stay updated, free articles. Join our Telegram channel
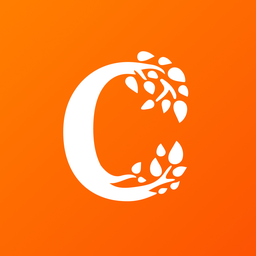
Full access? Get Clinical Tree
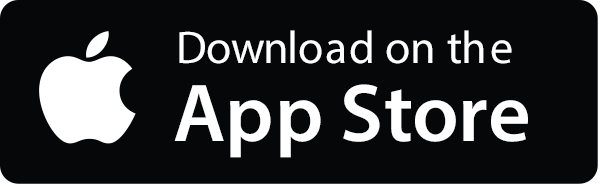
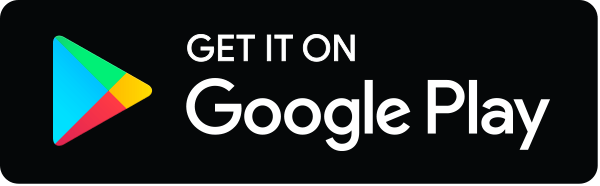
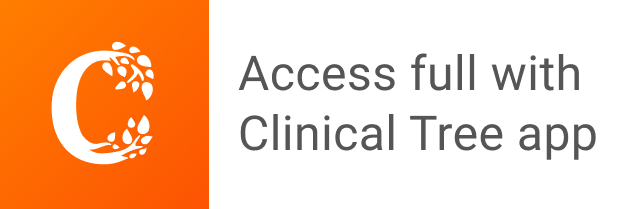