Carnitine1
Charles J. Rebouche
1Abbreviations: CoA, coenzyme A; Na+, sodium.
HISTORICAL OVERVIEW
Carnitine was first discovered in muscle extracts, independently by Gulewitsch and Krimberg and by Kutscher, in 1905, and the correct structure was assigned in 1927 by Tomita and Sendju (1). Between 1948 and 1952, Fraenkel et al demonstrated the essential nature of this compound for the mealworm, Tenebrio molitor, and assigned the term “vitamin BT” to carnitine (1). The role of carnitine in fatty acid oxidation was discovered independently by Bremer, and by Fritz and Yue, between 1962 and 1963 (2). The origin of the methyl groups of carnitine was identified by Wolf and Berger and by Bremer in 1961 (2), and the origin of the carbon chain of carnitine from the essential amino acid lysine was first reported by Tanphaichitr et al in 1971 (3). Clinical syndromes associated with carnitine deficiency were first reported by Engel et al in 1973 (4) and 1975 (5), and primary systemic carnitine deficiency associated specifically with a defect in carnitine transport was identified by Treem et al in 1988 (6).
CHEMISTRY AND NOMENCLATURE
L-Carnitine[R(-)-β-hydroxy-γ-(N,N,N-trimethylammonio) butyrate] is a zwitterionic quaternary amino acid (Fig. 32.1) with a molecular weight of 161.2 g/mol (inner salt). Only the L isomer is biologically active. L-Carnitine is present in biologic systems in both nonesterified and esterified forms. Short-chain (C2-C5) organic acids or medium (C6-C12)- or long (C14-C24)-chain fatty acids are transferred to and from coenzyme A (CoA) and the hydroxyl group of carnitine (see Fig. 32.1). These reversible reactions are catalyzed by a group of enzymes appropriately named carnitine acyltransferases.
DIETARY SOURCES
Carnitine is abundant in most food products of animal origin. Among all dietary sources, red meats contain the highest concentrations of carnitine. Fruits, vegetables, grains, and other plant-derived foods contain relatively little carnitine (7, 8). Thus, a normal omnivorous diet provides approximately 2 to 12 µmol/kg body weight/day of carnitine, whereas a strict vegetarian diet contains approximately 0.1 µmol/kg body weight/day (7).
REQUIREMENTS AND RECOMMENDED INTAKES
Carnitine is not a required nutrient for children and adults. No recommended dietary allowance has been established. Population groups that may be most vulnerable to nutritional carnitine deficiency are strict vegetarians and newborn infants. Strict vegetarians (both adults and children) acquire very little carnitine in their diets. They have somewhat lower plasma carnitine concentrations compared with omnivores, but no indication of clinically relevant carnitine deficiency has been reported (9).
Infants, particularly premature infants, are born with relatively low stores of carnitine, and rapid growth places a demand for carnitine accretion. In the past, but not currently, commercial soy protein-based infant formulas did not contain carnitine. Infants consuming these carnitinefree formulas grew at a normal rate and displayed no evidence of clinical carnitine deficiency, although some biochemical parameters relating to lipid metabolism (e.g., plasma free fatty acid concentration, rate of excretion of
medium-chain dicarboxylic acids) were different, compared with infants consuming the same formulas but supplemented with carnitine (10). An expert panel commissioned by the US Food and Drug Administration’s Center for Food Safety and Applied Nutrition recommended a minimum carnitine content in infant formulas of 7.5 µmol/100 kcal, and a maximum level of 12.4 µmol/100 kcal, a value similar to the upper limit reported for human milk (11). These recommendations were made on the basis of reported biochemical differences when infants were fed carnitine-free diets, compared with similar diets with carnitine, and despite the lack of evidence that carnitine is essential for the term infant.
medium-chain dicarboxylic acids) were different, compared with infants consuming the same formulas but supplemented with carnitine (10). An expert panel commissioned by the US Food and Drug Administration’s Center for Food Safety and Applied Nutrition recommended a minimum carnitine content in infant formulas of 7.5 µmol/100 kcal, and a maximum level of 12.4 µmol/100 kcal, a value similar to the upper limit reported for human milk (11). These recommendations were made on the basis of reported biochemical differences when infants were fed carnitine-free diets, compared with similar diets with carnitine, and despite the lack of evidence that carnitine is essential for the term infant.
HOMEOSTATIC MECHANISMS
Carnitine homeostasis in humans is maintained by the dynamic interactions of endogenous synthesis, acquisition from dietary sources, maintenance of concentration gradients across cell membranes, and regulation of renal reabsorption and excretion of carnitine.
Absorption and Bioavailability
Absorption of carnitine likely involves a combination of active transport and passive diffusion across the intestinal mucosal barrier. Evidence has accumulated from in vivo and in vitro studies, using several experimental preparations, to demonstrate that active transport of carnitine occurs across the apical brush-border membrane of enterocytes, but not across the basal membrane (9). A passive component, at least at the serosal surface, is strongly suggested by experimental studies in rats (12, 13) and Caco-2 cells (14) that demonstrated relatively rapid entry of carnitine into enterocytes from the luminal medium, but very slow appearance in the serosal perfusate or medium.
Approximately 63% to 75% of carnitine is absorbed from the normal omnivorous diet (15). The remainder is almost entirely degraded by bacteria in the large intestine. Primary organic degradation products of carnitine are trimethylamine (excreted in urine as trimethylamine oxide) and γ-butyrobetaine (excreted primarily in feces). Carnitine is not degraded by enzymes of animal origin (16).
Biosynthesis
Humans are able to synthesize carnitine from the essential amino acids lysine and methionine (Fig. 32.2). Lysine provides the carbon chain and nitrogen atom, and three methionine molecules (as S-adenosyl-L-methionine) provide the methyl groups for one molecule of carnitine (17). Methylation of the epsilon (ε) amino group of lysine is catalyzed by one or more protein:lysine methyltransferases. Lysine residues destined for carnitine synthesis must be peptide linked; no evidence indicates that free lysine is enzymatically methylated in mammals. &U20AC;-N-Trimethyllysine is released for carnitine synthesis through normal mechanisms of protein hydrolysis.
ε-N-Trimethyllysine undergoes four sequential enzymatic reactions (17): hydroxylation at position two of the carbon chain, catalyzed by ε-N-trimethyllysine hydroxylase (EC 1.14.11.8); aldol cleavage between carbons two and three of the carbon chain, catalyzed by serine hydroxymethyltransferase (EC 2.1.2.1); oxidation of the resulting aldehyde by any of several oxidized nicotinamide adenine dinucleotide (NAD1)-requiring aldehyde dehydrogenases (including one with high specificity for γ-N-trimethylaminobutyraldehyde); and a second hydroxylation, catalyzed by γ-butyrobetaine hydroxylase (EC 1.14.11.1). cDNAs coding for each of these four enzymes have been cloned and sequenced (18). All enzymes in the pathway except γ-butyrobetaine hydroxylase are ubiquitous in mammalian tissues. The last enzyme in the pathway is not found in cardiac or skeletal muscle (17). γ-Butyrobetaine hydroxylase activity is highest in liver and testes. In some species, including humans, it is abundant in kidney.
The normal rate of carnitine synthesis in humans is approximately 1.2 µmol/kg body weight/day (7). This estimate was obtained from normal rates of urinary carnitine excretion by strict vegetarians, who acquire very little carnitine (˜0.1 µmol/kg body weight/day) from dietary sources. Direct measurement of the rate of carnitine synthesis technically is not feasible (7). The problems of isotope dilution (only a very small percentage of the body lysine pool is used for carnitine synthesis) and uniform mixing within the body pool are overwhelming and preclude direct measurement of rates of carnitine synthesis from lysine. Direct determination of rates of carnitine synthesis
from ε-N-trimethyllysine by isotopic conversion is also impractical because this precursor does not readily cross cell membranes, and thus pools of intracellular free ε-N-trimethyllysine cannot be labeled uniformly.
from ε-N-trimethyllysine by isotopic conversion is also impractical because this precursor does not readily cross cell membranes, and thus pools of intracellular free ε-N-trimethyllysine cannot be labeled uniformly.
![]() Fig. 32.2. Pathway of carnitine biosynthesis in mammals. (Adapted with permission from Rebouche CJ. Ascorbic acid and carnitine biosynthesis. Am J Clin Nutr 1991;54(Suppl):1147S-52S.) |
The rate of carnitine synthesis in mammals is regulated by the availability of ε-N-trimethyllysine, which, in turn, is determined by the extent of peptide-linked lysine methylation and by the rate of protein turnover (7). ε-N-Trimethyllysine destined for carnitine synthesis probably is derived from the general protein pool and not from any single or small group of proteins. Provision of excess lysine in the diet may increase carnitine synthesis modestly (19), but the evidence is indirect, and the mechanism (e.g., increased flux through protein synthesis, methylation and turnover, or stimulation of a putative vestigial capability to methylate free lysine) has not been identified. The rate of carnitine biosynthesis does not appear to be affected by the magnitude of dietary carnitine intake or by changes in renal handling of carnitine.
Transport and Excretion
Carnitine is concentrated in most tissues of the body. In humans, the intracellular concentrations of carnitine in skeletal muscle and liver are approximately 76 and 50 times higher, respectively, than that in extracellular fluid (˜50 µmol/L). Approximately 97% of all carnitine in the body is in skeletal muscle. Six carnitine transporters have been identified: three organic cation transporters OCTN1, OCTN2, and OCTN3; a carnitine transporter, CT2; an organic anion transporter, Oat9S; and an amino acid transporter, ATB0,+.
OCTN1 is expressed in many tissues (although not in human adult liver) (20), but it has relatively low affinity (translocation rate constant, Kt = 412 µM) and specificity for carnitine (21). This pH-dependent, 63-kDa transporter may be responsible for secretion of carnitine and its short-chain esters across the renal epithelial brush-border membrane (22, 23). Carnitine is transported into most tissues by a high-affinity (Kt = 3 to 5 µM), sodium (Na+) gradient-dependent organic cation transporter, OCTN2 (24, 25). This 63-kDa transporter is highly expressed in the heart, placenta, skeletal muscle, kidney, pancreas, testis, and epididymis (20, 25) and weakly expressed in brain, lung, and liver (26). OCTN2 binds carnitine, acetylcarnitine, and propionylcarnitine with comparable affinity (27). It is likely to be quantitatively the most important carnitine transporter in all tissues except testis.
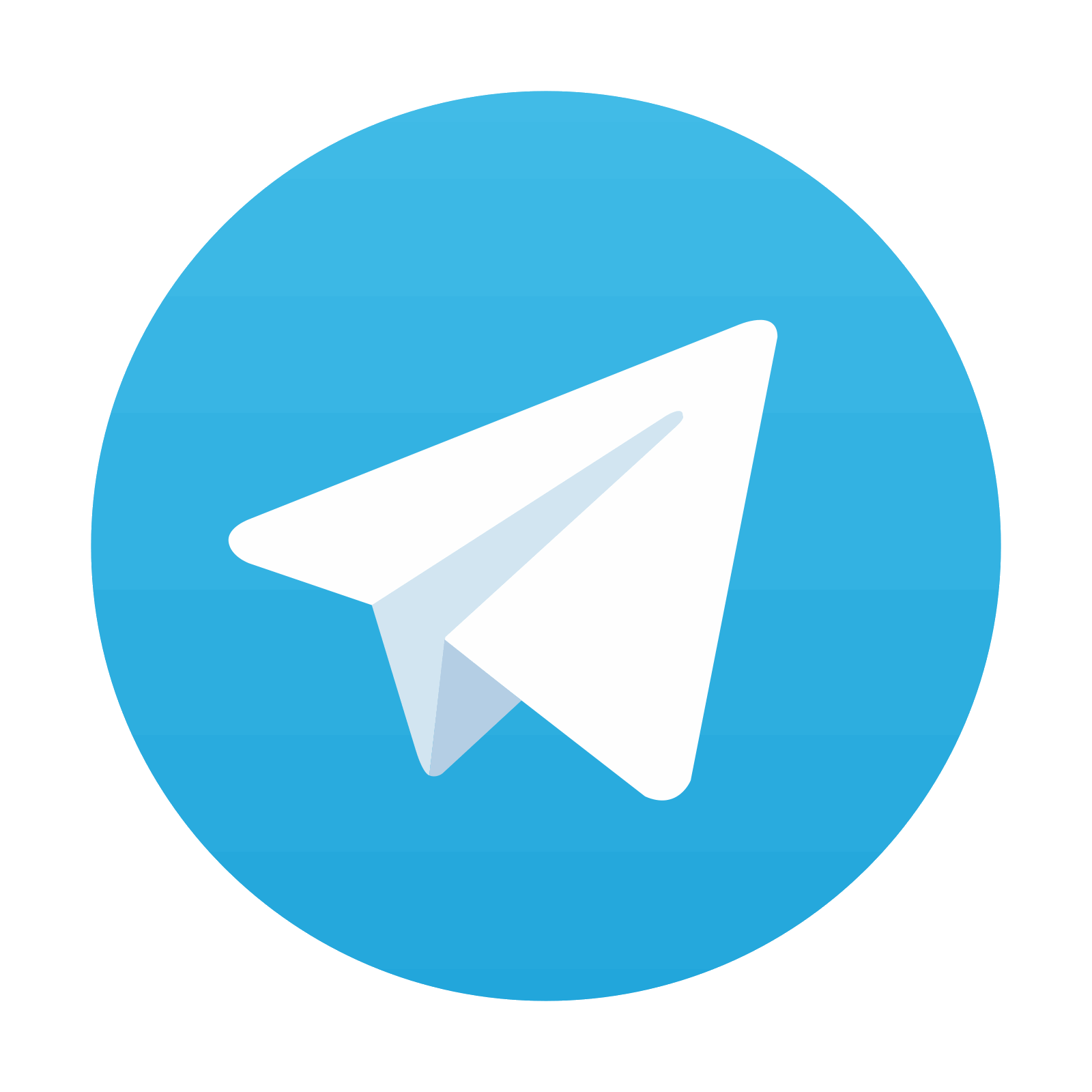
Stay updated, free articles. Join our Telegram channel
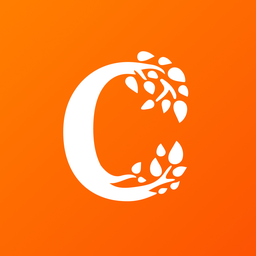
Full access? Get Clinical Tree
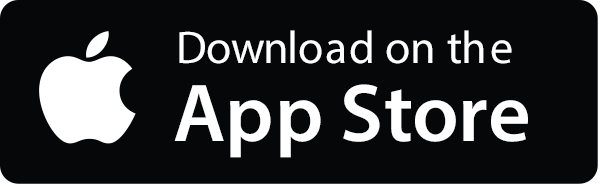
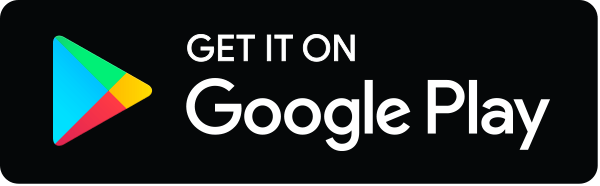