12 Carbohydrates
Introduction
The word ‘carbohydrate’ is used to encompass a large group of compounds with the general formula Cn(H2O)n. While the role of carbohydrates in cellular metabolism has been known for many years, carbohydrates have been more recently implicated in a wide range of cellular functions including protein folding, cell adhesion, enzyme activity, and immune recognition (Varki et al. 1999). Histochemical techniques for the detection and characterization of carbohydrates and carbohydrate-containing macromolecules (glycocongugates) are common practices in the histology laboratory. These techniques often provide invaluable information which may aid the pathologist in diagnosing and characterizing various pathological conditions including neoplasia, inflammation, autoimmune disorders, and infectious diseases.
Classification of carbohydrates
Carbohydrates are broken down into two broad categories: simple carbohydrates or those molecules composed purely of carbohydrates, and glycoconjugates, those molecules composed of carbohydrates and other molecules such as protein or lipid (Table 12.1). The simple carbohydrates are further categorized as monosaccharides, oligosaccharides, or polysaccharides. Glycoconjugates may further be broken down into proteoglycans, mucins, and ‘other’ glycoproteins. Although lipid-carbohydrate complexes are widely distributed in cells and tissues, these types of molecule are not discussed here, as they are not detectable by the routine histochemical techniques described in this chapter.
Table 12.1 Basic classification of carbohydrates and glycoconjugates
Simple carbohydrates |
Glycoconjugates |
Monosaccharide, the basic carbohydrate structure
The most basic or simple form of a carbohydrate is the monosaccharide. Typical monosaccharides are of the empirical formula (CH2O)n, where n is a value between 3 and 9. The basic monosaccharide is the six-carbon simple carbohydrate glucose (Fig. 12.1). Glucose is not charged or ionized and for this reason is referred to as a neutral sugar. Other neutral sugars include mannose, galactose, and fructose. Monosaccharides contain asymmetric carbons referred to as chiral centers. The letters D or L at the beginning of a name refer to the conformation of one of the chiral carbons within the molecule. This is of little interest to the reader with the exception that monosaccharides of the D conformation predominate in nature.
Polysaccharides
A polysaccharide is a large macromolecule composed of multiple monosaccharides joined by covalent bonds referred to as glycosidic linkages. Figure 12.2 demonstrates a α1–4 glycosidic linkage connecting molecules of glucose in a large polysaccharide. The α1–4 glycosidic linkage of glucose units is the predominant linkage in the polysaccharides starch and glycogen. In addition, some of the glucose units of these polysaccharides may be involved in more than one glycosidic linkage, thus forming a branching type of structure which may resemble a tree. Both glycogen and starch consist of glucose units with α1–4 as well as α1–6 glycosidic linkages, differing only in size and branching structure. Starch and glycogen are extremely large macromolecules with molecular weights that surpass 1 x 106 daltons.
There are a number of disease processes or pathological conditions in which histochemical assessment of glycogen content or accumulation may be of value diagnostically (Table 12.2). There are several well-characterized glycogen storage diseases which are the result of inherited defects of one or more of the enzymes involved in the synthesis or breakdown of glycogen (Cori & Cori 1952; Hers 1963). In most of these disorders, the liver shows massive accumulation of glycogen. In some diseases, glycogen accumulation is also observed in skeletal and cardiac muscle.
Connective tissue glycoconjugates – the proteoglycans
The carbohydrate components of proteoglycans are known as glycosaminoglycans. Glycosaminoglycans are large polysaccharide polymers that are covalently bound to the protein core of proteoglycans. The typical glycosaminoglycan is a long unbranched polysaccharide composed of repeating disaccharide units each made up of two different monosaccharides. Each disaccharide typically is composed of a carboxylated uronic acid (glucuronic or iduronic acid) and a hexosamine such as N-acetyglucosamine or N-acetylgalactosamine. The hexosamines frequently contain highly acidic sulfate groups. There are six distinct classes of glycosaminoglycans (Table 12.3). The chondroitin sulfates are the most abundant of the glycosaminoglycans in the human. Figure 12.3a illustrates the structure of the repetitive disaccharide unit of chondroitin 4-sulfate.
Table 12.3 Characterization of glycosaminoglycans
Glycosaminoglycan | Disaccharide repeat | Location |
---|---|---|
Chondroitin sulfatea | Glucuronic acid and N-acetylgalactosamine | Cartilage, tendons, ligaments, aorta, cell membranes |
Dermatan sulfate | Iduronic acid and N-acetylgalactosamine | Skin, blood vessels, heart valves |
Keratan sulfate | Galactose and N-acetylglucosamine | Cartilage, cornea |
Heparin sulfateb | Glucuronic acid and N-acetylglucosamine or N-sulfate glucosamine | Blood vessels, aorta, cell membranes |
Heparin | Glucuronic acid and N-acetylglucosamine or N-sulfate glucosamine | Mast cell granules |
Hyaluronic acid | Glucuronic acid and N-acetylglucosamine | Synovial fluid, vitreous humor, loose connective tissues Small amounts are found in cartilage where it serves as a scaffold for the proteoglycans |
a Chondroitin sulfates may be subcategorized as chondroitin 4-sulfate or chondroitin 6-sulfate depending upon the position of the sulfate group in the N-acetylgalactosamine.
b Heparin and heparin sulfate differ structurally in the degree of sulfation of the glucosamine units. Heparin contains more sulfate and fewer N-acetyl groups in this unit.
The glycosaminoglycan chains are covalently bound to a protein core of the proteoglycan via the side chain of the amino acids serine or threonine (O-glycosidic linkage) and to a lesser extent to asparagine (N-glycosidic linkage). The number of glycosaminoglycan chains varies greatly among different proteoglycans. An exception to this structural motif is hyaluronic acid, which does not contain a covalently bound protein core (Mason et al. 1982).
Hyaluronic acid (Fig. 12.3b) is a polymer of repeating N-acetylglucosamine and glucuronic acid disaccharide units (Roden 1980). Hyaluronic acid also differs from the other glycosaminoglycans in the absence of sulfate groups. In spite of these differences, hyaluronic acid is classified as a glycosaminoglycan because of its overall structural similarity to the other glycosaminoglycans.
The negatively charged sulfate and/or carboxyl groups together with numerous hydroxyl groupings render most proteoglycans extremely hydrophilic. This property accounts for the gel-like consistency of the extracellular matrix and connective tissues such as cartilage. The proteoglycans of cartilage in particular contain many glycosaminoglycan chains and thus are capable of binding a large volume of water. Proteoglycans act in stabilizing and supporting fibrous elements of connective tissue. Tissues that contain high concentrations of proteoglycans include cartilage, tendons, ligaments, blood vessels, heart valves, and skin (Table 12.3). Hyaluronic acid is found in high concentrations in synovial fluid and in the ground substance and connective tissue matrices where other proteoglycans are found.
Several pathological conditions involve accumulation of glycosaminoglycans or proteoglycans (Table 12.2). The mucopolysaccharidoses are a group of genetic disorders that result from a deficiency of one or more of the enzymes that are involved in the degradation of heparin sulfate and dermatan sulfate (McKusick & Neufeld 1983). This results in the abnormal accumulation of glycosaminoglycans in connective tissues as well as cell types such as neurons, histiocytes, and macrophages.
Glycosaminoglycans and proteoglycans are expressed by a number of different sarcomas. Hyaluronic acid and chondroitin sulfates in particular may be found in high concentrations in myxoid chondrosarcomas as well as the myxoid variants of liposarcoma and malignant fibrous histiocytoma (Tighe 1963; Kindblom & Angervall 1975; Weiss & Goldblum 2001). In addition, proteoglycans may be observed in the stromal components of sarcomas as well as some carcinomas.
Mucins
Mucins, like the proteoglycans, consist of polysaccharide chains covalently linked to a protein core (Gendler & Spicer 1995). Typically, the carbohydrate component is attached via an O-glycosidic linkage to serine or threonine. The serine and threonine-rich protein core may contain anywhere from several hundred to several thousand amino acids. A defining structure of the epithelial mucins is the presence of tandemly repeated amino acid sequences within the protein core. Mucins are categorized into functionally distinct families (muc1, muc2, muc3, etc.) based in part upon differences in the amino acid sequences within the tandem repeats and the structure of their protein core (Perez-Vilar & Hill 1999).
The sialic acids (Fig. 12.4) are a diverse group of nine-carbon monosaccharides that contain a carboxylate group at the carbon in position 1 (Schauer 1982; Varki et al. 1999). The carboxylate group is ionized at a physiological pH and imparts an overall negative charge on the molecule.
The function of the mucins depends in part upon the tissue location of the mucin-producing cell as well as the mucin type. In most cases, the secreted mucins provide lubrication and protection for the secreting cells and/or tissues in the immediate area. The function or role of the membrane-bound mucins is not well understood. These mucins are likely involved in the regulation of cellular functions such as cell proliferation and cell adhesion (Wesseling et al. 1995; Moniaux et al. 1999; Schroeder et al. 2001).
While immunohistochemistry has largely replaced special stains in the differential diagnosis of anaplastic tumors or tumors of unknown origin, detection of mucin in a tumor may be a valuable clue in the identification of a malignancy. Malignancies derived from simple epithelial tissues (carcinomas) frequently contain detectable mucin. In contrast, melanomas, lymphomas, and sarcomas rarely exhibit significant levels of mucins. In addition, determining the type of mucin (i.e. neutral or acidic) may be helpful in evaluating neoplastic changes within a tissue. The detection of acid or sulfomucins within the gastric mucosa may aid in the detection and characterization of intestinal metaplasia, a lesion associated with gastric carcinoma (Turani et al. 1986).
Fixation
The selection of an appropriate fixative for the histochemical detection of carbohydrates depends largely on the type of carbohydrate to be demonstrated. The fixation of glycogen is somewhat more demanding than that of the glycoconjugates such as the mucins and proteoglycans. Due to the aqueous solubility of glycogen, many of the older studies recommended the avoidance of aqueous-based fixatives such as formalin (Lillie 1954). It is now accepted that glycogen loss during formalin fixation usually does not compromise the ability to detect glycogen with techniques such as the periodic acid-Schiff (PAS) method. This is likely due to the retention of a portion of the cellular glycogen by non-covalent association with adjacent proteins (Manns 1958).
While neutral buffered formalin (NBF) is an acceptable fixative for glycogen, there is agreement that alcoholic formalins are superior fixatives for glycogen preservation (Lillie 1954). Rossman’s fluid (alcoholic formalin with picric acid) has also been recommended for glycogen fixation (Bancroft & Cook 1994). Mercuric chloride containing fixatives such as Zenker’s-acetic acid or Susa’s are not recommended for fixation of glycogen-containing tissues (Manns 1958; Bancroft & Cook 1994).
Regardless of the fixative used, it is essential that tissues intended for glycogen analysis should be placed in a fixative promptly following removal. Glycogen present in many animal tissues is extremely labile to autolytic changes. If immediate fixation is not possible, the tissue should be refrigerated until adequate fixation in possible. When possible, fixation should be carried out at 4°C to minimize the streaming artifact which frequently occurs in fixed tissues (Lillie 1954).
The fixation requirements for the mucins and proteoglycans are less stringent than for glycogen. As these carbohydrates are covalently bound to proteins, the principal effect of fixation occurs on the protein portion of the molecule. In most cases, formalin or alcoholic formalin fixation is adequate for preservation. The carbohydrate deposits of the mucopolysaccharidoses have been reported to be less stable than typical mucins or proteoglycans (Bancroft & Cook 1994). In these cases, fresh or frozen sections are recommended although alcoholic formalin is also satisfactory.
Techniques for the demonstration of carbohydrates
The histochemical techniques used for the demonstration of carbohydrates and glycoconjugates are outlined in (Table 12.4). In the text that follows the potential mechanism(s) for each technique is discussed. Emphasis also is placed on the specificity of the techniques.
Table 12.4 Summary of the specificity of the techniques for the detection of carbohydrates and glycoconjugates

The periodic acid-Schiff (PAS) technique
The PAS technique is without question the most versatile and widely used technique for the demonstration of carbohydrates or glycoconjugates. The first histochemical use of this technique was by McManus (1946) for the demonstration of mucin. Subsequently other studies have demonstrated the utility of the PAS technique for demonstration of other carbohydrate-containing molecules, such as glycogen and certain glycoproteins (Lillie 1947, 1951; McManus 1948). The list of PAS-reactive tissues and cell types is long and varied. Table 12.5 is a listing of the PAS-reactive tissues, cells, and cellular structures that are commonly evaluated in the histology laboratory. This listing is not intended to be all-inclusive and the reader is directed to several references (Lillie 1951; Thompson 1966; Bancroft & Cook 1994) for more extensive data.
Table 12.5 PAS-reactive cells and tissue components
The PAS technique may aid in the differential diagnosis of tumors through the detection of mucins or glycogen. The reactivity of Schiff reagent with glycoproteins within the basal lamina makes the PAS technique a valuable means of assessing basement membrane thickness (Hennigar 1987). Increased basement membrane thickness, particularly in the glomerular capillaries of the kidney, is indicative of a number of pathological conditions. The PAS technique also is a sensitive and relatively fast means of demonstrating viable fungi in tissue sections. This is due to the presence of periodic acid-reactive polysaccharides in the capsules or walls of many fungal species. Common fungal species that are PAS reactive include Candida albicans, Histoplasma capsulatum, Cryptococcus, and Blastomyces (Harley 1987).
Mechanism of the PAS technique
As typically employed in the histology laboratory, the PAS technique is based upon the reactivity of free aldehyde groups within carbohydrates with the Schiff reagent to form a bright red/magenta end product. The initial step in the PAS technique is the oxidation of hydroxyl groups attached to adjacent carbon atoms (1,2-glycols) within the carbohydrate. The result is the formation of two free aldehyde groups and the cleavage of the adjoining carbon-to-carbon bond (Fig. 12.5). The oxidation of the 1,2-glycols to form adjacent aldehydes is produced by treatment of the sections with a dilute solution of periodic acid (HIO4). In most protocols a 0.5–1.0% solution of periodic acid is used for 5–10 minutes. Other oxidants such as chromic acid and potassium permanganate have been used in variations of the technique (Bauer 1933; Thompson 1966). However, these oxidants tend further to oxidize the aldehyde groups to carboxylic groups which are not reactive with Schiff reagent. As a result, the sensitivity of techniques using these oxidants is less than that of the PAS technique.
The intensity of the color that develops following reaction with Schiff reagent is dependent upon the tissue concentrations of reactive glycol structures (Leblond et al. 1957). Monosaccharides that lack 1,2-glycols or contain hydroxyl groups that are involved in an ester or glycosidic linkage are not susceptible to periodic acid oxidation and hence cannot be detected with the PAS technique.
Periodic acid also is known to oxidize substance other than carbohydrates to form reactive aldehydes. The α-amino alcohols of serine and threonine are oxidized by periodic acid but only when present at the end of the protein chain (Thompson 1966). It is also possible for periodic acid to oxidize hydroxylysine regardless of the position in the protein chain. However, it is doubtful whether the reactivity of these amino acids contributes significantly to the PAS reactivity in tissue sections.
Preparation of Schiff reagent
Schiff reagent is prepared from basic fuchsin. Basic fuchsin is not a specific dye but instead represents a mixture of triarylmethane dyes such as pararosaniline, rosaniline, and new fuchsin (Lillie 1977). The individual components of basic fuchsin also provide satisfactory starting points for the preparation of Schiff reagent.
A number of methods for the synthesis of Schiff reagent have been described since Schiff’s original report in 1866. All of these methods share a common theme in the production of an aqueous solution of sulfurous acid. The sulfurous acid may be generated from the reaction of sodium metabisulfite (Na2S2O5) with a mineral acid such as hydrochloric acid (HCl), or by the reaction of thionyl chloride (SOCl2) with water (Barger & DeLamater 1948; Longley 1952). Sulfur dioxide is the active agent in the production of Schiff reagent and the source of the sulfur dioxide is not critical as long as by-products of the reaction to produce sulfurous acid do not interfere with the reaction of sulfurous acid with basic fuchsin (Barger & DeLamater 1948).
The reaction of sulfur dioxide with basic fuchsin results in the addition of a sulfonic acid group to the central carbon of the triarylmethane molecule. The magenta or red coloration is lost due to the reduction of the quinoid configuration within the triarylmethane molecule. The free amino groups of the triarylmethane react with additional one or two equivalents of sulfur dioxide to form Schiff reagent (Lillie 1977). As described above, Schiff reagent reacts with the free aldehydes generated from 1,2-glycol groups in periodic acid-treated carbohydrates. The initial monosaccharide-Schiff reagent conjugate is a colorless reaction intermediate. The loosely bound sulfonate of the central carbon is removed in a subsequent aqueous rinse. The re-establishment of the quinoid structure of the triarylmethane molecule results in the deposition of a deep red/magenta coloration in the site of the carbohydrate-Schiff reagent complex (Lillie 1977).
PAS technique (modified McManus 1946)
Periodic acid solution
Periodic acid | 1 g |
Distilled water | 100 ml |
Method
1. Dewax in xylene and rehydrate through graded ethanols to distilled water.
2. Oxidize with periodic acid for 5 minutes.
3. Rinse in several changes of distilled water.
4. Cover the sections with Schiff reagent for 15 minutes.
5. Rinse in running tap water for 5–10 minutes.
6. Stain the nuclei with hematoxylin. Differentiate and blue the sections.
7. Dehydrate in graded ethanols and clear with xylene.
Notes
a. The intensity of stain is dependent to some extent on the length of treatment with the periodic acid and Schiff reagent. For basement membranes, a longer time in periodic acid (10 minutes) and Schiff reagent (20 minutes) may give better results.
b. Earlier descriptions of the PAS procedure frequently recommended post-Schiff bisulfite rinses for the reduction of background. This is not necessary provided the slides are adequately rinsed in tap water.
c. Fixatives containing glutaraldehyde should be avoided if tissues are to be stained with the PAS technique. This is because glutaraldehyde contains two aldehyde groups; tissues fixed in glutaraldehyde contain free aldehyde groups capable of undergoing the Schiff reaction. This results in non-specific background staining.
d. Staining of glycolipids may be detected when frozen sections are used. In addition, staining of unsaturated lipids may occur in some cases due to the oxidation of carbon-to-carbon double bonds to produce Schiff-reactive aldehyde groups. However, glycolipids and unsaturated lipids rarely interfere with interpretation of results obtained from paraffin-embedded tissues as a significant loss of these molecules likely occurs during tissue processing.
Alcian blue
Standard alcian blue technique
Alcian blue is a large conjugated dye molecule that initially was used for the dyeing of textile fibers. It is composed of a central copper-containing phthalocyanine ring linked to four isothiouronium groups via thioether bonds (Scott et al. 1964). The isothioronium groups are moderately strong bases and account for the cationic nature of alcian blue (Scott et al. 1964). A variety of different alcian blue dyes have been produced in the past that differ in the number of linked isothiouronium groups as well as the components of the diluent (Scott et al. 1964; Horobin & Kiernan 2002). Alcian blue 8GX is the recommended dye for histological techniques (Scott & Mowry 1970).
While the exact mechanisms by which alcian blue stains carbohydrates are unknown, it is widely believed that the cationic isothiouronium groups bond via electrostatic linkages with polyanionic molecules within tissues (Pearse 1960; Quintarelli et al. 1964). The sulfate and carboxylate groups of chondroitin sulfate, dermatan sulfate, heparin sulfate, and hyaluronic acid are ionized at a pH of 2.5 and therefore carry a negative charge. This accounts for the staining of the proteoglycan/hyaluronic acid components of connective tissue and cartilage with alcian blue at a pH of 2.5. Similarly, the acidic epithelial mucins such as the sialomucins and sulfomucins of the large intestine are reactive at pH 2.5. Neutral mucins such as those in the gastric mucosa and Brunner’s glands are not reactive with alcian blue.
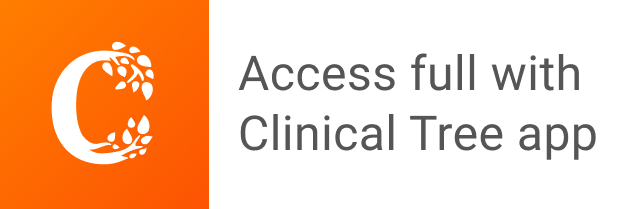