Present evidence indicates that approximately one in four deaths is now due to cancer and that invasive cancer will be diagnosed in more than half of the population at some point in their lives. Many cancers are increasing in incidence, largely as a result of the increased average age of our population.
As shown in this chapter, the causes of cancer are a mixture of environmental and genetic alterations that occur in our tissues. Inherited predisposition plays a role in some families. Dramatic advances in molecular biology and genetics have now clarified the basic molecular elements of cancer and provide a schematic outline of the cellular events leading to cancer. This understanding will be crucially important in the control of cancer, providing the beginnings of a base of knowledge that should lead to significantly improved therapies and preventive strategies.
“Cancer” is a collection of disorders that share the common feature of uncontrolled cell growth. This leads to a mass of cells termed a neoplasm (Greek, “new formation”), or tumor. The formation of tumors is called tumorigenesis. Several key events must occur if cells are to escape the usual constraints that prevent uncontrolled proliferation. Additional growth signals must be produced and processed, and cells must become resistant to signals that normally inhibit growth. Because these abnormal characteristics would typically trigger the process of programmed cell death (apoptosis), cells must somehow disable this process. The growing cell mass (tumor) requires nourishment, so a new blood supply must be obtained through angiogenesis (the formation of new blood vessels). Additional inhibitory signals must be overcome for the tumor to achieve a malignant state, in which neoplasms invade nearby tissues and metastasize (spread) to more distant sites in the body. The capacity to invade and metastasize distinguishes malignant from benign neoplasms.
Tumors are classified according to the tissue type in which they arise. Major types of tumors include those of epithelial tissue ( carcinomas, the most common tumors), connective tissue (sarcomas), lymphatic tissue (lymphomas), glial cells of the central nervous system (gliomas), and hematopoietic organs (leukemias). The cells that compose a tumor are usually derived from a single ancestral cell, making them a single clone (monoclonal).
Many of the basic biological features of carcinogenesis (cancer development) are now understood. Throughout our lives, many of our cells continue to grow and differentiate. These cells form, for example, the epithelial layers of our lungs and colons and the precursor cells of our immune systems. Relatively undifferentiated stem cells produce large numbers of progeny cells to repopulate and renew our worn defensive layers. Through integration of information provided by a complex array of biochemical signals, the new cells eventually stop dividing and terminally differentiate into a cell type appropriate for their role in the body ( Fig. 11.1 ). Alternatively, if the cell is abnormal or damaged, it may undergo apoptosis.

Occasionally one of these cells fails to differentiate and begins to divide without restraint. The descendants of such cells can become the founders of neoplasms, capable of further transformation into invasive, metastatic cancer. We wish to understand in detail what has gone wrong in these cells, to detect them early, and ultimately to intervene in their development so as to eliminate them.
Cells in the body are programmed to develop, grow, differentiate, and die in response to a complex system of biochemical signals. Cancer results from the emergence of a clone of cells freed of these developmental programming constraints and capable of inappropriate proliferation.
Causes of Cancer
Genetic Considerations
Genetic alterations of cell regulatory systems are the primary basis of carcinogenesis. We can create cancer in animal models by damaging specific genes. In cell culture systems, we can reverse a cancer phenotype by introducing normal copies of the damaged genes into the cell. Most of the genetic events that cause cancer occur in somatic cells. The frequency of these events can be altered by exposure to mutagens, thus establishing a link to environmental carcinogens (cancer-causing agents). However, these genetic events are not transmitted to future generations because they occur in somatic, rather than germline, cells. Even though they are genetic events, they are not inherited.
It is also possible for cancer-predisposing mutations to occur in germline cells. This results in the transmission of cancer-causing alleles from one generation to the next, producing families that have a high incidence of specific cancers ( Fig. 11.2 ). Such “cancer families,” although rare, demonstrate that inheritance of a damaged gene can cause cancer. In these families, inheritance of one mutant allele seems sufficient to cause a specific form of cancer. Most individuals who inherit the mutant allele will develop a tumor. This is because each of their cells now carries the altered gene and thus has already taken the first step down the cancer pathway. The childhood cancer of the eye, retinoblastoma, is a good example. As discussed in Chapter 4 , those who inherit a mutant version of the retinoblastoma gene have approximately a 90% chance of developing one or more retinoblastoma tumors.

Although the transmission of cancer as a single-gene disorder is relatively uncommon, there is good evidence for more frequent clustering of some cancer types in families. For many kinds of cancer, such as those of the breast, colon, and prostate, the diagnosis of the cancer in a first-degree relative implies a two- to three-fold increase in one’s risk of developing the cancer. The inheritance of altered forms of specific genes is at least partly responsible for this increased risk.
The extent to which each of these mechanisms—inherited germline mutations versus mutations occurring in somatic cells—contributes to human cancer is an important question. If inherited predispositions are significant determinants of a person’s risk of acquiring a specific form of cancer, it should be possible to identify those in whom the risk is elevated. More intensive screening of defined high-risk groups can result in early detection and intervention, leading to better prognoses for patients and lowered morbidity and mortality.
The basic cause of cancer is damage to specific genes. Usually mutations in these genes accumulate in somatic cells over the years, until a cell accumulates a sufficient number of errors to initiate a tumor. If damage occurs in cells of the germline, however, an altered form of one of these genes can be transmitted to progeny and predispose them to cancer. The increased risk of cancer in such persons is due to the fact that each of their cells now carries the first step in a multistep cancer pathway.
Environmental Considerations
What is the role of the nongenetic environment in carcinogenesis? At the level of the cell, cancer seems intrinsically genetic. Tumor cells arise when certain changes, or mutations, occur in genes that are responsible for regulating the cell’s growth. However, the frequency and consequences of these mutations can be altered by a large number of environmental factors. It is well documented, for example, that many chemicals that cause mutation in experimental animals also cause cancer and are thus carcinogens. Furthermore, other environmental agents can enhance the growth of genetically altered cells without directly causing new mutations. Thus it is often the interaction of genes with the environment that determines carcinogenesis; both play key roles in this process.
Two additional lines of argument support the idea that exposure to environmental agents can significantly alter a person’s risk of cancer. The first is that a number of environmental agents with carcinogenic properties have been identified. For example, epidemiological studies and laboratory experiments have shown that cigarette smoke causes lung and other types of cancer. Roles for other environmental agents in specific cancers are also well documented (e.g., uranium dust in lung cancer among miners, asbestos exposure in lung cancer and mesothelioma).
The second line of argument is based on epidemiological comparisons among populations with differing lifestyles. Many kinds of cancer have quite different frequencies in different populations. Breast cancer, for example, is prevalent among northern Europeans and European Americans, but relatively rare among women in developing countries. It is usually difficult to determine whether such dissimilarities reflect differences in lifestyle or in gene frequencies.
Examination of genetically similar populations under differing lifestyles, however, provides an opportunity to evaluate the genetic and environmental components of cancer. Epidemiological studies among migrant Japanese populations have yielded important findings with respect to colon cancer. This type of cancer was, until recently, relatively rare in the Japanese population living in Japan, with a lifetime risk of 0.5%, but it is 10 times more common in the United States. Stomach cancer, on the other hand, is common in Japan but relatively rare in the United States. These statistics in themselves cannot distinguish environmental from genetic influences in the two populations. However, because large numbers of Japanese have immigrated, first to Hawaii and then to the U.S. mainland, we can observe what happens to the rates of stomach and colon cancer among the immigrants. It is important that many of the Japanese immigrants maintained a genetic identity, marrying largely among themselves. Among the first-generation Japanese in Hawaii, the incidence of colon cancer rose several-fold—not yet as high as in the U.S. mainland, but higher than in Japan. Among second-generation Japanese Americans on the U.S. mainland, the colon cancer rate rose to 5%, equal to the U.S. average. At the same time, stomach cancer has become relatively rare among Japanese Americans.
These observations strongly suggest an important role for environment or lifestyle in the etiology of colon cancer. In each case, diet is a likely culprit; a high-fat, low-fiber diet in the United States is thought to increase the risk of colon cancer, whereas techniques used to preserve and season the fish commonly eaten in Japan are thought to increase the risk of stomach cancer. It is also interesting that the incidence of colon cancer in Japan has increased dramatically during the past several decades, as the Japanese population has adopted a diet more similar to that of North America and Europe.
Are we then to assume that genetic factors play no role in colon cancer? The fact remains that in the North American environment some people will get colon cancer but most will not. This distinction can result from differences within this environment (e.g., dietary variation) as well as differences in genetic predisposition: inherited genes that increase a person’s probability of developing cancer. To account for the difference in colon cancer incidence between Japanese living in the U.S. and those living in Japan, it is argued that environmental features in Japan render the predisposing genes less penetrant. Furthermore, a genetic component is strongly suggested by the several-fold increase in risk to a person when a first-degree relative has colon cancer. It is likely, then, that cancer risk is a composite of both genetics and environment, with interaction between the two components.
Environmental factors are known to play important roles in carcinogenesis. However, a person’s overall cancer risk depends on a combination of inherited factors and environmental components.
Cancer Genes
Genetic Control of Cell Growth and Differentiation
Cancers form when a clone of cells loses the normal controls over growth and differentiation. More than 100 cancer-causing genes that encode proteins participating in this regulation have now been identified. Characterization of the biochemical activities and interactions of these gene products has revealed an increasingly detailed picture of the normal regulation of cell growth and differentiation and the ways these processes become deregulated by the events of carcinogenesis.
Many features of this fundamental process are now well understood ( Fig. 11.3 ). One component of cell regulation is mediated by external signals coming to the cell through growth factors (e.g., platelet-derived growth factor, epidermal growth factor, steroid hormones) produced in other cells. Each growth factor interacts with specific growth factor receptors located on the cell surface. Binding of a growth factor activates the receptor, triggering molecules that send messages to the cell’s nucleus in the process of signal transduction. These signal transduction molecules include protein kinases, such as Src tyrosine kinase, mitogen-activated protein kinase (MAPK), and Jun kinase (JunK), which can alter the activity of target proteins by tagging them at a specific site with a phosphate molecule (phosphorylation). The ultimate stage of the signal transduction pathway is regulation of DNA transcription in the nucleus. Components of the signal transduction cascade interact with nuclear transcription factors that regulate the activity of specific genes whose protein products influence cellular growth and proliferation. Genes that encode these transcription factors include MYC, FOS, and JUN .

After several rounds of cell division, cells normally receive signals that tell them to stop growing and to differentiate into specialized cells. The signals may come from polypeptides, from steroid hormones, from direct contact with adjacent cells, or from internal programs that define the number of cell divisions that are allowed. The signals are transduced to the nucleus of the recipient cell. Here, by altering the transcription patterns of genes that govern the steps of the cell cycle, they repress genes that promote cell division and induce genes that inhibit entry into the cell division cycle.
The regulation of cell growth is accomplished by substances that include (1) growth factors that transmit signals from one cell to another; (2) specific receptors for the growth factors; (3) signal transduction molecules that activate a cascade of phosphorylating reactions within the cell; and (4) nuclear transcription factors. The cell integrates and interprets the host of signals it receives from its environment. Decisions to grow and divide, or to stop growing and differentiate, result from processing of these signals.
A cancer cell can emerge from within a population of growing cells through the accumulation of mutations in these genes. Although such mutations occur only rarely, these cells can fail to respond to differentiation signals and continue to divide instead of undergoing their normal differentiation program. Furthermore, cancers usually seem to result from a progressive series of events that incrementally increase the extent of deregulation within a cell lineage. Eventually a cell emerges whose descendants multiply without appropriate restraints. Further changes give these cells the capacity to invade adjacent tissues and form metastases. Each of these changes involves mutations, and the requirement for more than one mutation has been characterized as the multi-hit concept of carcinogenesis. An example of this concept is given by colorectal cancer, in which several genetic events are required to complete the progression from a benign growth to a malignant neoplasm (see later discussion).
Mutations can occur in any of the steps involved in regulation of cell growth and differentiation. Accumulation of such mutations within a cell lineage can result in a progressive deregulation of growth, eventually producing a tumor cell.
The Inherited Cancer Gene Versus the Somatically Altered Gene
Although “cancer families” have long been recognized, it was not until the early 1970s that we began to understand the relationship between the inherited genetic aberrations and the carcinogenic events that occur in somatic tissue. In 1971 Alfred Knudson’s analysis of retinoblastoma, a disease already mentioned as a model of inherited cancer, led him to a hypothesis that opened a new window into the mechanism of carcinogenesis. In the inherited form of retinoblastoma (see Chapter 4 ), an affected individual often has an affected parent, and there is a 50% chance of genetic transmission to each of the offspring. In the sporadic (noninherited) form, neither parent is affected, nor is there additional risk to other progeny. A key feature distinguishing the two forms is that inherited retinoblastoma is usually bilateral (affecting both eyes), whereas sporadic retinoblastoma usually involves only a single tumor and therefore affects only one eye (unilateral).
Knudson reasoned that at least two mutations may be required to create a retinoblastoma. One of the mutations would alter the retinoblastoma gene; if this happened in the germline, it would be present in all cells of a child who received the mutant allele. The second mutation would be an additional, unspecified genetic event occurring in an already-altered cell. The hypothesis of a second event was required to explain why only a tiny fraction of the retinoblasts of a person who has inherited a mutant retinoblastoma gene actually give rise to tumors. Knudson’s hypothesis is known as the two-hit model of carcinogenesis.
Familial retinoblastoma would thus be caused by the inheritance of one of the genetic “hits” as a constitutional mutation (i.e., a mutation present in all cells of the body). Persons who inherited one hit would require only one additional mutational event in a single retinoblast for that cell to seed a tumor clone. In sporadic cases, on the other hand, both mutations would have to occur somatically in the developing fetus ( Fig. 11.4 ). This is a highly improbable combination of rare events, even considering the several million cells of the target tissue. The child who developed a retinoblastoma by this two-hit somatic route would be unlikely to develop more than one tumor. The child inheriting a mutant retinoblastoma gene, however, would need only a single, additional genetic hit in a retinoblast for a tumor clone to develop. Knudson argued that such an event was likely to occur in several of the retinoblasts of each carrier of the inherited mutant gene, thus explaining the bilaterality of inherited retinoblastoma.

If the first event in the two-hit model can be an inherited mutation, what is the nature of the second hit? Extensive molecular analysis of the region of chromosome 13 that contains the retinoblastoma-causing gene, RB1, showed that the second hit, like the first one, is a loss-of-function mutation. Several mechanisms, including point mutation, deletion, and hypermethylation of the RB1 promoter region (associated with decreased transcription; see Chapter 3, Chapter 5 ), can produce this effect. The second hit, which occurs in the fetus during the period in which retinoblasts are rapidly dividing and proliferating, has removed the remaining normal allele of this gene. This implies that a cell with one mutant RB1 allele and one normal RB1 allele cannot form a tumor. Thus the product of the normal gene, even when present only in a single copy, prevents tumor formation.
An important corollary of this two-hit hypothesis is that the genes in which inherited mutations cause familial cancer syndromes may be the same as those that generate common cancers by somatic mutation. Therefore by understanding the nature of the mutant alleles inherited in rare cancer families, we will come to understand more about the somatic pathway to common cancer as well. Indeed, somatic loss-of-function mutations of both copies of the RB1 gene are seen frequently in many types of tumors, including small-cell lung carcinoma, breast carcinoma, glioblastoma (a brain tumor), and osteosarcoma.
Alfred Knudson’s two-hit theory of carcinogenesis in retinoblastoma became the paradigm for a model to describe how inheritance of an altered gene predisposes the gene carrier to cancer. The theory states that a cell can initiate a tumor only when it contains two damaged alleles; therefore a person who inherits one copy of a mutant retinoblastoma gene must experience a second, somatic mutation in one or more retinoblasts in order to develop one or more retinoblastomas. Two somatic mutations can also occur in a single retinoblast of a nonpredisposed fetus, producing sporadic retinoblastoma. Understanding mutated genes that are inherited in families can increase our understanding of the somatic pathway to common cancers.
Major Classes of Cancer Genes
Cancer-causing genes can be classified into three major categories: those that normally inhibit cellular proliferation (tumor suppressors), those that activate proliferation (oncogenes), and those that participate in DNA repair.
Tumor Suppressor Genes
The RB1 gene was the first identified example of a tumor suppressor gene, a class of genes that control cell division and thus help to prevent tumors ( Table 11.1 ). A perplexing feature of tumor suppressor genes is that inherited mutations are dominant alleles at the level of the individual (i.e., heterozygotes usually develop the disease), but they are recessive alleles at the level of the cell (heterozygous cells do not form tumors). This apparent contradiction is resolved by realizing that in individuals who have inherited the first hit, a second hit that occurs in any one cell will cause a tumor. Because there are several million target retinoblasts in the developing fetus, heterozygous persons form, on the average, several retinoblasts homozygous for an RB1 mutation. Each of these can lead to a retinoblastoma. Thus it is the strong predisposition to tumor formation (i.e., the first hit) that is inherited as an autosomal dominant trait. The incomplete penetrance of the retinoblastoma mutation (90%) is explained by the fact that some people who inherit the disease-causing mutation do not experience a second hit in any of their surviving retinoblasts.
Gene (Related Genes in Parentheses) | Function of Gene Product | Disease Caused by Germline Mutations |
---|---|---|
Tumor Suppressor Genes | ||
RB1(p107, p130) | Cell cycle brake; binds to E2F transcription factor complex | Retinoblastoma; osteosarcoma |
APC | Interacts with β-catenin in Wnt signaling pathway | Familial adenomatous polyposis |
SMAD4 | Transmits signals from TGFβ | Juvenile polyposis |
NF1 | Down-regulates RAS protein | Neurofibromatosis type 1 |
NF2 | Cytoskeletal protein regulation | Neurofibromatosis type 2 |
TP53 | Transcription factor; induces cell cycle arrest or apoptosis | Li–Fraumeni syndrome |
VHL | Regulates multiple proteins, including p53 and NFκB | Von Hippel–Lindau disease (renal cysts and cancer) |
WT1 | Zinc finger transcription factor; binds to epidermal growth factor gene | Wilms tumor |
CDKN2A (p14, p16) | CDK4 inhibitor | Familial melanoma |
PTEN | Phosphatase that regulates PI3K signaling pathway | Cowden syndrome (breast and thyroid cancer) |
CHEK2 | Phosphorylates p53 and BRCA1 | Li–Fraumeni syndrome |
PTCH | Sonic hedgehog receptor | Gorlin syndrome (basal cell carcinoma, medulloblastoma) |
CDH1 | E-cadherin; regulates cell–cell adhesion | Gastric carcinoma |
DPC4 | Transduces transforming growth factor-β signals | Juvenile polyposis |
TSC2 | Down-regulates mTOR (mammalian target of rapamycin) | Tuberous sclerosis |
DNA Repair Genes | ||
MLH1 | DNA mismatch repair | HNPCC |
MSH2 | DNA mismatch repair | HNPCC |
BRCA1 | Interacts with BRCA2/RAD51 DNA repair protein complex | Familial breast and ovarian cancer |
BRCA2 | Interacts with RAD51 DNA repair protein | Familial breast and ovarian cancer |
ATM | Protein kinase; phosphorylates BRCA1 in response to DNA damage | Ataxia telangiectasia; conflicting evidence for direct involvement in breast cancer |
XPA | Nucleotide excision repair | Xeroderma pigmentosum |
A general property of tumor suppressors is that they normally block the uncontrolled cellular proliferation that can lead to cancer. Often this is done by participating in pathways that regulate the cell cycle. For example, the protein encoded by RB1 (pRb) is active when it is unphosphorylated, but is down-regulated when it is phosphorylated by cyclin-dependent kinases (CDKs) just before the S phase of the cell cycle (see Chapter 2 ). In its active, hypophosphorylated state, pRb binds to members of the E2F transcription complex, inactivating them ( Fig. 11.5 ). E2F activity is required for the cell’s progression into S phase, so its inactivation by pRb halts the cell cycle. Thus pRb serves as a cell-cycle brake that is normally released only when pRb is inactivated through phosphorylation by CDKs. This allows the cell to proceed through its mitotic cycle until pRb is activated again through removal of the phosphate groups. A loss-of-function mutation in RB1, a deletion of the gene, or hypermethylation of its 5′ region can lead to its permanent inactivation. Without this brake on the cell cycle, the cell can proceed through numerous uncontrolled divisions.

Loss-of-function mutations of other inhibitory factors can also lead to an unregulated cell cycle. A number of tumor suppressor genes encode CDK inhibitors (see Fig. 11.5 ), which inactivate CDKs and thus prevent them from phosphorylating target proteins such as pRb. Tumor suppressors can also control cell proliferation through their effects on transcription or on cell–cell interactions (some examples are discussed later). Again, mutations in these genes can lead to unrestricted cell division and ultimately to cancer.
The discovery that retinoblastoma results when both alleles of the same locus on chromosome 13 are inactivated in the same retinoblast led to the concept of tumor suppressor genes. The products of such genes suppress tumor formation by controlling cell growth and can do so even if a cell contains only one normal version of the gene. Loss-of-function mutations that inactivate both copies of a cell’s tumor suppressor gene can lead to uncontrolled cellular proliferation.
Because of the pivotal role of tumor suppressors in preventing tumor formation, their study is of considerable medical significance. By understanding how cancer is naturally suppressed by the body, we can ultimately develop more effective medical therapies for tumor prevention and treatment.
Oncogenes
Oncogenes (i.e., “cancer genes”) are a second category of genes that can cause cancer. Most oncogenes originate from proto-oncogenes, which are genes involved in the four basic regulators of normal cell growth mentioned previously (growth factors, growth factor receptors, signal transduction molecules, and nuclear transcription factors). When a mutation occurs in a proto-oncogene, it can become an oncogene, a gene whose excessively active product can lead to unregulated cell growth and differentiation. When a cell proceeds from regulated to unregulated growth, the cell is said to have been transformed.
Unlike tumor suppressor genes, oncogenes are usually dominant at the cellular level; only a single copy of a mutated oncogene is required to contribute to the multistep process of tumor progression. Whereas tumor suppressors are typically disabled by deletions or loss-of-function mutations, oncogenes are typically activated by gain-of-function mutations, gene amplification (i.e., increased numbers of the gene through trisomy or other mechanisms), hypomethylation of the oncogene’s 5′ region (which increases transcription), or chromosome rearrangements that up-regulate the oncogene (e.g., the Philadelphia chromosome translocation, Chapter 6 ). Most tumor suppressor genes are known to exhibit germline mutations that can cause inherited cancer syndromes (e.g., retinoblastoma, Li–Fraumeni syndrome). In contrast, although oncogenes are commonly found in sporadic tumors, germline oncogene mutations that cause inherited cancer syndromes are uncommon (a few exceptions are noted later in the discussion). These and other differences between tumor suppressor genes and oncogenes are summarized in Table 11.2 .
Feature | Tumor Suppressor Genes | Oncogenes |
---|---|---|
Function of normal version | Regulates cell growth and proliferation; some can induce apoptosis | Promotes cell growth and proliferation |
Mutation (at cell level) | Recessive (both copies of gene inactivated) | Dominant (only one copy of gene mutated) |
Effect of mutation | Loss of function | Gain of function |
Germline mutations resulting in inherited cancer syndromes | Seen in most tumor suppressor genes | Seen in only a few oncogenes |
In this section, we review three approaches that have been used to identify specific oncogenes: retroviral definition, transfection experiments, and mapping in tumors.
Proto-oncogenes encode products that control cell growth and differentiation. When mutated or amplified, they can become oncogenes, which can cause cancer. Most oncogenes act as dominant gain-of-function mutations that lead to the deregulation of cell cycle control. In contrast to tumor suppressor genes, most oncogenes do not exhibit germline mutations that cause inherited cancer syndromes. Instead, somatic mutations are seen that lead to sporadic cancers.
It has long been known that certain types of viruses can cause cancer. Especially significant are retroviruses, a type of RNA virus that is capable of using reverse transcriptase to transcribe its RNA into DNA. In this way the RNA genome of the retrovirus is converted to DNA, which can be inserted into a chromosome of a host cell. Some retroviruses carry altered versions of growth-promoting genes into cells. These growth-promoting genes are oncogenes, which were first identified through the study of retroviruses that cause cancer in chickens. When the retrovirus invades a new cell, it can transfer the oncogene into the genome of the new host, thus transforming the cell and initiating cancer.
A number of gene products that affect cell growth or differentiation have been identified through the study of oncogenes carried by transforming retroviruses. For example, retrovirus studies identified the gene encoding the receptor molecule for epidermal growth factor (EGF), through the ERBB oncogene. These studies also identified the RAS ( ra t s arcoma) oncogenes, which are altered in at least 25% of human cancers. Transforming retroviruses have also identified the nuclear transcription factor genes, MYC, JUN, and FOS, as other molecular components capable of initiating cell transformation. Table 11.3 provides additional examples of proto-oncogenes.
Oncogene | Function | Associated Tumor |
---|---|---|
Growth Factor Genes | ||
HST | Fibroblast growth factor | Stomach carcinoma |
SIS | β subunit of platelet-derived growth factor | Glioma (brain tumor) |
KS3 | Fibroblast growth factor | Kaposi sarcoma |
Growth Factor Receptor Genes | ||
RET † | Receptor tyrosine kinase | Multiple endocrine neoplasia; thyroid carcinoma |
ERBB | Epidermal growth factor receptor | Glioblastoma (brain tumor); breast carcinoma |
ERBA | Thyroid hormone receptor | Acute promyelocytic leukemia |
NEU (ERBB2) | Receptor protein kinase | Neuroblastoma; breast carcinoma |
MET † | Receptor tyrosine kinase | Hereditary papillary renal carcinoma; hepatocellular carcinoma |
KIT † | Receptor tyrosine kinase | Gastrointestinal stromal tumor syndrome |
Signal Transduction Genes | ||
HRAS | GTPase | Carcinoma of colon, lung, pancreas |
KRAS | GTPase | Melanoma, thyroid carcinoma, acute monocytic leukemia, colorectal carcinoma |
NRAS | GTPase | Melanoma |
BRAF | Serine/threonine kinase | Malignant melanoma; colon cancer |
ABL | Protein kinase | Chronic myelogenous leukemia; acute lymphocytic leukemia |
CDK4 † | Cyclin-dependent kinase | Malignant melanoma |
Transcription Factor Genes | ||
NMYC | DNA-binding protein | Neuroblastoma; lung carcinoma |
MYB | DNA-binding protein | Malignant melanoma; lymphoma; leukemia |
FOS | Interacts with JUN oncogene to regulate transcription | Osteosarcoma |
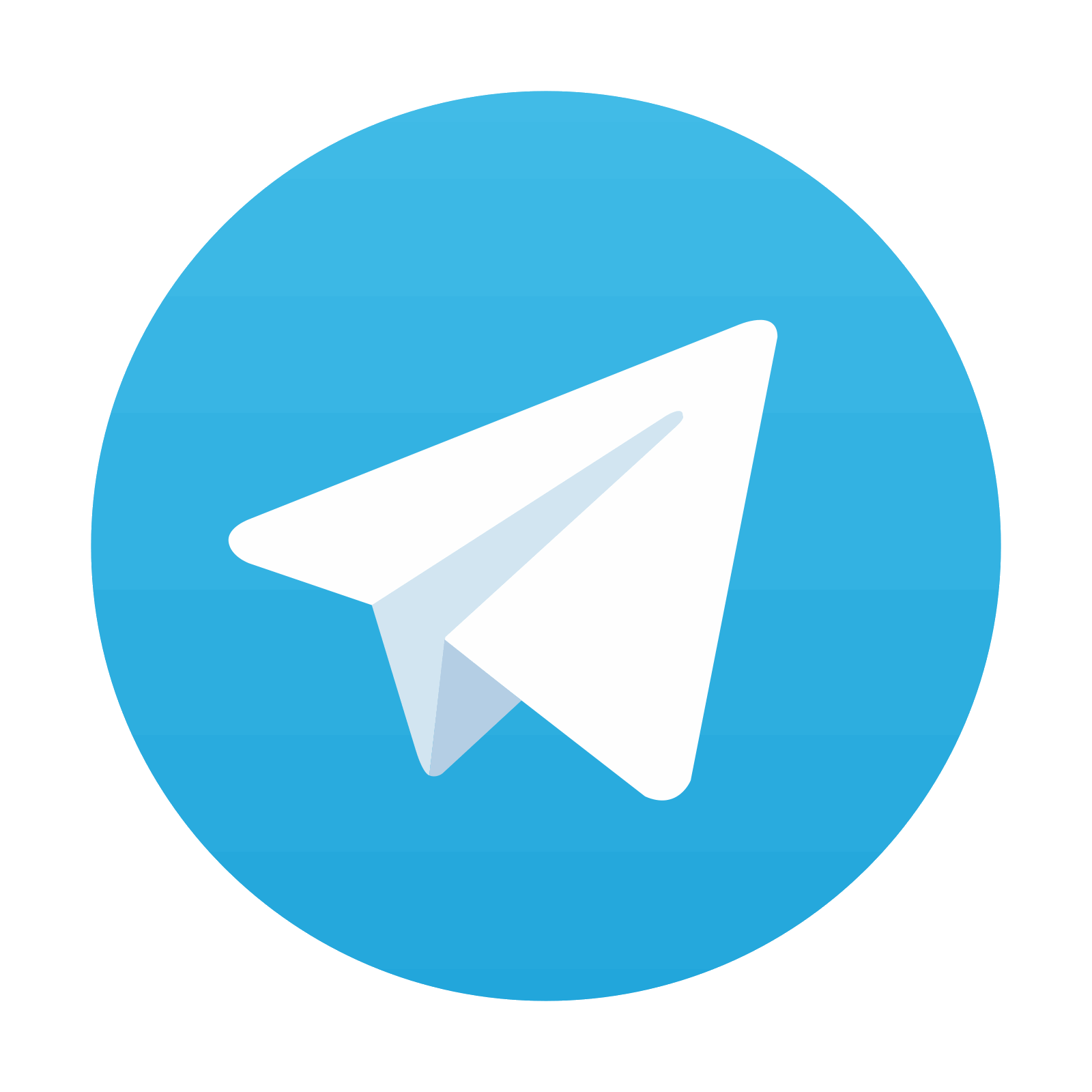
Stay updated, free articles. Join our Telegram channel
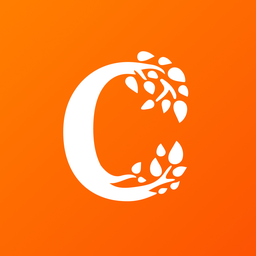
Full access? Get Clinical Tree
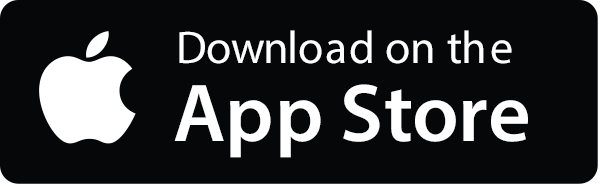
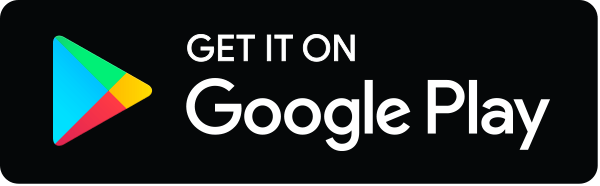
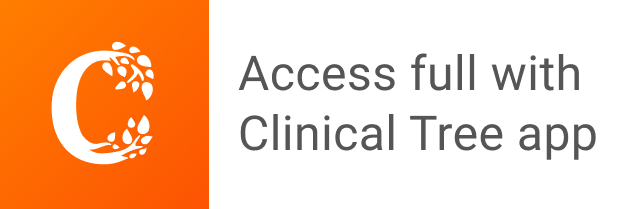