Cancer Ablation: Understanding the Technologies and Their Applications
Salomao Faintuch
Muneeb Ahmed
S. Nahum Goldberg
Introduction
Thermal (radiofrequency [RF], microwave, laser, and cryoablation) and nonthermal (chemical and irreversible electroporation [IRE]) ablative therapies have gained increasing clinical acceptance as methods for treating focal primary and secondary malignancies of the liver, kidney, lung, and bone.
This chapter presents the general principles and underlying rationale for commonly used ablative tumor therapies. Recent technological advances and future directions will also be discussed.
Approaches to Tumor Ablation
The use of percutaneous image guidance facilitates the least invasive approach for needle-based tumor therapies. By using imaging such as ultrasound, computed tomography (CT), or magnetic resonance imaging (MRI), tumors can be precisely targeted and treated by advancing a percutaneous needle applicator. Only a few needle insertions are necessary, and no skin incision is created. Most of these procedures can be safely performed under local anesthesia and intravenous moderate sedation, on an outpatient basis.
Tumor ablations have also been successfully performed using laparoscopic and open surgical approaches, under general anesthesia, with varying degrees of invasiveness.
Objectives of Tumor Ablation
The primary goal of most ablation procedures is to eradicate all viable malignant cells within a designated target volume. Similar to the surgical approach of metastasectomy, tumor ablation therapies attempt to include at least a 0.5 to 1.0 cm ablative margin of seemingly normal tissue for the liver and the lung, though less may be needed for some tumors in the kidney. While complete tumor eradication is of primary importance, sparing normal surrounding tissues and accuracy of therapy are also important clinical considerations. As such, one significant advantage of percutaneous ablative therapies over conventional standard surgical resection is the potential to remove or destroy only a minimal amount of normal tissue. For example, in primary liver tumors, where functional hepatic reserve is a primary predictive factor in long-term patient survival outcomes, ablation therapies can minimize iatrogenic damage to surrounding cirrhotic parenchyma. This is also useful when nephron-sparing treatments are needed in patients with von Hippel-Lindau, who are prone to the development of multiple renal cell carcinomas, and in patients with primary lung malignancies in the setting of extensive underlying emphysema and limited lung function. Other clinical circumstances in which high specificity and accuracy of targeting have proven useful include providing symptomatic relief for patients with symptomatic osseous metastases or hormonally active neuroendocrine tumors, and in using percutaneous therapies to improve focal interstitial drug delivery. Percutaneous thermal therapies are limited, however, by the quality of imaging guidance, and in some cases, by complex anatomy and difficult access. Another important factor is that the amount of tumor destruction is determined by the pattern of temperature, energy, or chemical distribution within treated tissues. This means that for larger tumors (usually defined to be larger than 3 cm in diameter), a single ablation treatment may not be sufficient to entirely encompass the desired ablative margins. In these instances, multiple overlapping ablations or simultaneous use of multiple applicators may be required to successfully treat the entire tumor and ablative margin, though accurate targeting and applicator placement can often be technically challenging in this scenario.
Techniques for Tumor Ablation
Chemical Ablation
Ethanol instillation has been used mainly for treating hepatocellular carcinoma in patients with cirrhosis. Ethanol destroys tissue by causing dehydration of the cytoplasm and protein denaturation. Furthermore, alcohol entering the local tumor circulation leads to necrosis of the vascular endothelium, subsequent vascular thrombosis, and ultimately ischemic tissue necrosis. The treatment of primary hepatocellular carcinoma with ethanol injection has been considerably more successful than the treatment of liver metastases because of tumor characteristics, including softer tissue composition compared to the heterogeneous and dense fibrous nature of metastases, and a capsule or pseudo-capsule surrounded by cirrhotic liver, which limits diffusion and increases concentration within the target (Fig. 1).
Intratumoral administration of chemically ablative substances such as ethanol and acetic acid is the ablation modality that has the longest experience and clinical follow-up, particularly in the treatment of hepatocellular carcinoma. Chemical ablation is an attractive option in many developing regions because it is inexpensive and simple. However, success of chemical ablation therapies in more solid adenocarcinomas has been limited by reported difficulty in achieving uniform diffusion of percutaneously injected drugs over larger tumor volumes, due to poor diffusion of chemical agents throughout the tumor. As a result, focal thermal ablation has replaced chemical ablation in many cases. Chemical ablation is now more typically used for tumors difficult to treat with thermal therapies (mostly due to tumor proximity to heat sensitive adjacent structures), and for small tumors such as locally recurrent thyroid cancer or benign lesions such as bronchogenic cysts, thyroglossal duct cysts, or endometriomas.
Thermal Ablation Therapies
Thermal ablation strategies attempt to destroy tumor tissue by increasing or decreasing temperatures sufficiently to induce irreversible cellular injury. These strategies can be broadly divided into cryoablation or hyperthermic ablation, where heat may be generated by ultrasound or electromagnetic (RF, microwave, and laser) energy. Other techniques to generate heat or otherwise induce cellular necrosis have been reported; however, none have found widespread utility to date.
Radiofrequency Ablation
By far, the most well-studied and clinically relevant percutaneous ablation source to date has been RF energy. During RF ablation, electrical current from the generator oscillates between electrodes through ion channels present in most biological tissues. In this way, the RF ablation setup can be thought of as a simple electrical circuit, where the current loop comprises a generator, cabling, electrodes, and tissues as the resistive element. Tissues are imperfect conductors of electricity (i.e., they have electrical impedance), so current flow leads to frictional agitation at the ionic level and heat generation, known as the Joule effect. Heating occurs most rapidly in areas of high current density; tissues nearest to an electrode are heated most effectively while more peripheral areas receive heat by thermal conduction.
Ablative heating leads to tissue dehydration and water vaporization, which cause dramatic increases in circuit impedance. These rapid and often sudden increases in impedance can be used as a feedback signal in RF generators, which will be covered in more detail later. When these effects begin to inhibit current flow from a generator, alternative methods to decrease circuit impedance such as expanding the electrode surface area, pulsing the input power, and injections of saline can be used to augment RF current flow.
Irreversible cellular injury occurs when cells are heated to 46°C for 60 minutes, and occurs more rapidly as the temperature rises. Immediate cellular damage centers on protein coagulation of cytosolic and mitochondrial enzymes and nucleic acid–histone protein complexes. The exact temperature at which cell death occurs is multifactorial and tissue specific. Based upon prior studies demonstrating that tissue coagulation can be induced by focal tissue heating to ∼50°C for <5 minutes, this has become the standard surrogate endpoint for thermal ablation therapies (Fig. 2).
Electrodes
Although any conductor and power source can create a thermal ablation, the power output and control algorithms of each generator have been tailored to suit their associated electrode(s). Most RF ablation systems today operate in a monopolar mode using two different types of electrodes: interstitial and dispersive electrodes (ground pads) on the skin surface. The interstitial electrode delivers energy to the tumor, creating a volume of high current density and localized heating. The ground pad closes the electrical current path, but is designed to disperse energy over a large surface area to reduce the likelihood of thermal injury to the skin.
Monopolar electrode designs include both straight insulated needles with an exposed metallic tip and multi-tined electrodes. Internally cooled electrodes use a single needle, in which fluid is circulated inside the electrode’s active tip, and temperatures at the electrode–tissue interface are reduced. Lower temperatures inhibit charring, which in turn allows increased power deposition. In effect, internal cooling drives RF heating from the electrode–tissue interface deeper into the tissue to create more clinically relevant ablations (∼2 cm in diameter in normal liver). When using water as a cooling fluid, the initial temperature of the water does not seem to impact device performance. The cooled needle design also uses a smaller caliber applicator (17-gauge, 1.5-mm diameter), compared to expandable electrodes. Internally cooled needles are now employed by the Cool-tip™ system (Covidien, Boulder, CO).
In contrast, electrodes with multiple tines emanating from a single electrode sheath or handle assembly aim to distribute energy spatially. The use of multiple tines improves heating efficiency in the target volume and also increases total electrode surface area, thereby reducing circuit impedance and promoting greater energy deposition. As a result, larger zones of ablation and potentially faster heating can be obtained. One type of multi-tined electrode utilizes three single 17-gauge electrodes, spaced 5 mm apart in a triangular configuration and driven in parallel by the same generator source. This configuration effectively behaves as a single, larger electrode but with a limited puncture area, and can create zones of ablation over 3 cm in diameter in normal liver in 12 minutes with a 200 W generator. The Cluster™ electrode (Covidien) is an example of a nondeployable multi-tined electrode.
Other multi-tined electrode designs deploy several smaller electrodes from a single needle shaft. Two such designs are clinically available today that create either star-shaped or umbrella-shaped arrays. Star-shaped electrodes are deployable from a 14-gauge (2.1-mm diameter) needle using arrays of 4, 9, or 12 tines. Many such electrodes also utilize hollow tines capable of injecting saline into the surrounding tissue to reduce impedance and increase energy delivery. Umbrella-shaped electrodes, on the other hand, contain 10 tines and are deployed from a 13-gauge needle. These tines are electrically connected and operated in parallel, which means that current flowing through each tine can vary depending upon local tissue properties. Deployable electrodes are capable of creating zones of ablation approximately 5 to 7 cm in diameter, though care should be taken when evaluating device performance since deployable designs have also been associated with irregular heating patterns. In general, multi-tined electrodes are more invasive and may increase complication rates, especially in percutaneous settings, though relevant comparisons between devices are lacking. Introduction or retraction complications with deployable electrodes have been reported, but are relatively rare. Examples of multi-tined deployable designs include the Starburst™ (RITA Medical Systems, Mountain View, CA) and LeVeen™ (Boston Scientific Corporation, Natick, MA) electrodes (Fig. 3).
Alternatively, in bipolar systems, current oscillates between two interstitial electrodes without the need for a ground pad. The electrodes may lie on separate applicators, or be situated longitudinally along the same applicator. The bipolar setup restricts current flow primarily to the area between the electrodes and protects this area from perfusion-mediated cooling, resulting in faster and more focal heating between the electrodes. Bipolar operation may require more precise placement of the electrodes to create a confluent zone of necrosis and can be limited by local changes in conductivity resulting from the ablation. For this reason, bipolar systems often use saline infusion to increase energy delivery between the electrodes. More recently, “multipolar” operation has become available, which involves switching between pairs of bipolar electrodes situated on individual needles. The ProSurge™ system (Celon AG;
Teltow, Germany) is an example of a multipolar device.
Teltow, Germany) is an example of a multipolar device.
Generator and Ground Pads
The RF ablation generator provides three essential functions: power generation, control, and user interface. Power output is controlled by the output voltage and circuit impedance, which includes all possible factors such as electrode design, target tissue environment, background tissue properties, and ground pad connectivity. Since power depends on impedance, RF ablation may be limited in areas of high background impedance, such as the lung, even if the tumor exhibits a relatively high conductivity. As tissue impedance rises, power output tends to decrease. Generators are now available from 150 to 250 W, which may be underpowered for some clinical scenarios (e.g., in lung). Higher-power designs are currently under development to address these problems.
The most important distinguishing factor between commercially available generators is in the feedback and control system. Both impedance-based and electrode temperature-based controls are available. In the Boston Scientific system, power is set initially at a relatively low level (typically 20 to 50 W) and gradually increased until impedance elevates to near-infinite levels. In the Covidien system, initial power is usually set to the maximum level. When the circuit impedance spikes rapidly, the generator turns off RF power for a short period to facilitate tissue cooling, which allows more power to be used when the generator switches back on. This power pulsing algorithm has been shown to increase ablation zone size and decrease treatment time.
Switching between multiple electrodes based on impedance spikes uses the inherent off-time of the power pulsing algorithm to deliver energy through another electrically independent electrode. Up to three equivalent ablations can be created independently in the same time as a single-electrode ablation, or a closely spaced array can be used to create large, conglomerate ablations with substantial time savings over the technique of overlapping single-electrode ablations.
Electrode tip temperatures are also monitored in some
systems; however, only the AngioDynamics/RITA system uses that information to control power output. In that system, generator output is ramped until electrode temperatures reach a predetermined value, usually around 95°C, and maintained at this temperature for typically 20 to 30 minutes. This power delivery strategy works effectively with multiple-tine electrode designs because of the dispersion in current density and large electrode surface area.
systems; however, only the AngioDynamics/RITA system uses that information to control power output. In that system, generator output is ramped until electrode temperatures reach a predetermined value, usually around 95°C, and maintained at this temperature for typically 20 to 30 minutes. This power delivery strategy works effectively with multiple-tine electrode designs because of the dispersion in current density and large electrode surface area.
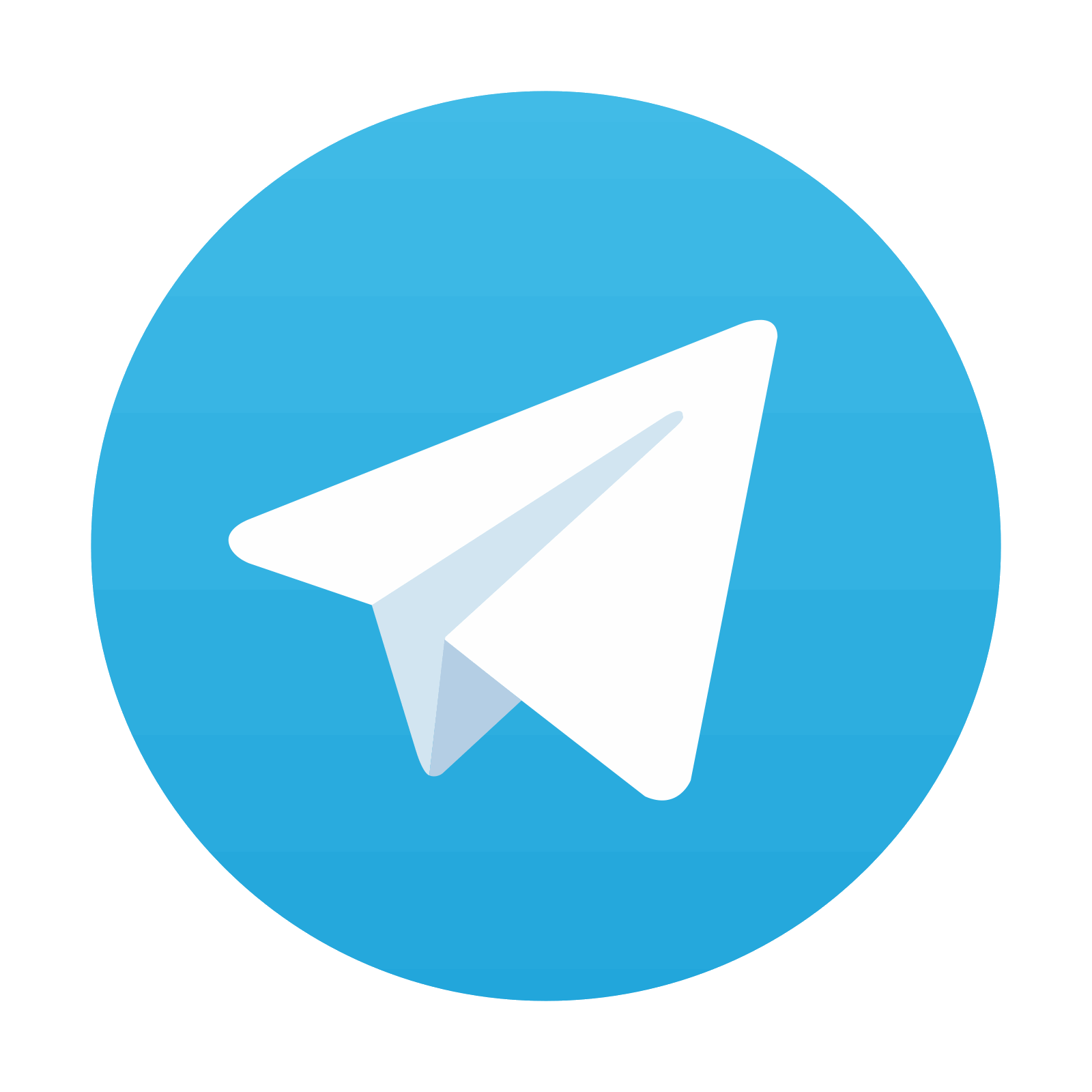
Stay updated, free articles. Join our Telegram channel
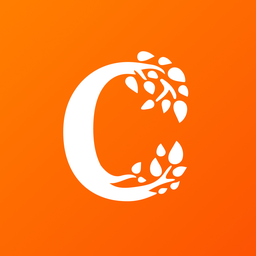
Full access? Get Clinical Tree
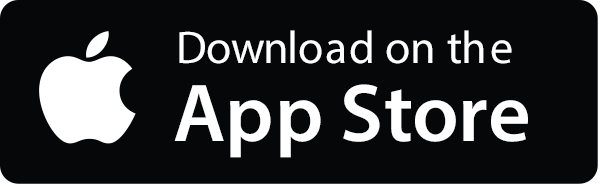
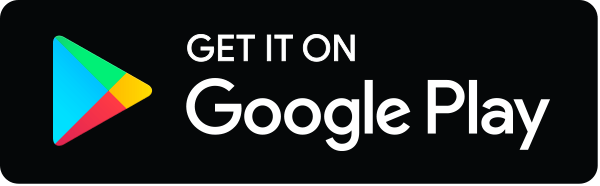
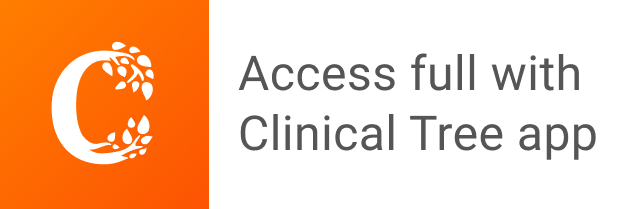