Bringing Research to Clinical Application Lessons from ThermoDox®: A Thermal-Sensitive Liposome for Treatment of Cancer |
CONTENTS
23.1 Initial Perspective and Scope: Where Are the Lessons to Be Learned?
23.1.1 In the Liposome Field Itself
23.1.2 Example of an Early “Lesson Learned” in Liposomes: Is It Being Heeded Today in Nanomedicine?
23.1.4 Early Commercialization and Access to a Wealth of Expertise: Just Ask Us
23.2.1 Doxorubicin and Liposomes
23.2.1.1 Doxorubicin, the Drug
23.2.1.2 Doxorubicin Encapsulated in Liposomes
23.2.1.3 New Toxicities for Doxil®
23.2.2.1 Effects of Hyperthermia
23.2.3 Lipid Membrane Mechanochemistry
23.2.3.1 Starting with Red Blood Cells
23.2.3.2 Giant Unilamellar Vesicle Experiments
23.3 Engineering Design of the LTSL
23.3.1 Lysolipid Exchange with Membranes Generates the “Idea”
23.4 Performance in Preclinical Studies
23.4.1 All 11/11 Mice Are “Cured”
23.4.2 Not All Cancers Respond the Same
23.4.4 ThermoDox® Phase 1 in Canine Patients
23.6.1 Phase 1 Prostate Cancer
23.6.2 Phase 2 Recurrent Chest Wall Cancer
23.6.2.1 RCW Protocol and ThermoDox® Pharmacokinetics
23.6.3 Phase 1 for Liver Cancer
23.6.4 Phase 3 for Liver Cancer HEAT Study (Hepatocellular Carcinoma Study of RFA and ThermoDox®)
23.6.4.1 Phase 3 Trial Fails to Meet 33% PFS
23.6.4.3 Phase 3 Trial Results
23.6.4.5 Second-Line Data Show Very Positive Results
23.6.6 Our Recommended Protocol
23.6.7 New and Ongoing Human Clinical Trials for ThermoDox®
23.7.2 Finally! Lessons Learned from ThermoDox®
23.8 New Directions: Put the Drug in the Cancer’s Food
23.9 Translating Drug Delivery without Profit: Open-Source Pharmaceutics?
23.1 INITIAL PERSPECTIVE AND SCOPE: WHERE ARE THE LESSONS TO BE LEARNED?
This is the story of our low-temperature-sensitive liposome (LTSL) (Needham et al. 2000), subsequently called ThermoDox® by the company Celsion Corporation which licensed it from Duke University in November 1999. It is an account of “lessons learned,” as told by me, as I saw it, experienced it, and lived it, and so much of this will be written in the first person. Everyone has their own story. I, myself, Celsion, and ThermoDox® have ours. As Bernard Malamud says in his book, The Natural, “We have two lives, the one we learn with, and the life we live after that” (Malamud 1952). This certainly applies to me and Celsion. So I write this not for Celsion, or for Duke, but for the next generation of inventors, carers, and entrepreneurs, who have a life to learn with and perhaps have yet to make their own mistakes or achieve their own real and verifiable successes. This chapter contains some of what you might have in store. I encourage you to heed these “lessons learned.” See if you can benefit from the positive results and avoid the ones that, let’s say, made life more difficult. At least be aware of what could happen if you, as the inventor, trust anyone else with your precious invention, which you will have to do. I know this is a long and detailed story, but please try and make it all the way to, what will hopefully be, a happy ending.
23.1.1 IN THE LIPOSOME FIELD ITSELF
There are always “lessons to be learned,” and the liposome field is no exception, especially with its event-rich and inextricably linked journey from research to commercialization. Although slightly before my own time in liposomes,* but not in research (Eley and Needham 1984; Needham 1981), the liposome field rapidly progressed in the mid- to late 1970s. The challenge for the early pioneers was to identify where this seemingly high potential impact, biocompatible, emerging clinical technology could, in fact, find its therapeutic application. Immunology and especially anticancer drug encapsulation and targeting took the center stage. Thus, once liposomes had been “discovered” (Bangham and Horne 1964), much of this pioneering work was led by luminaries such as Dimitri Papahadjopoulos and Gregory Gregoriadis and included reports on making and characterizing liposomes in terms of structure and permeability (Papahadjopoulos and Miller 1967; Papahadjopoulos and Watkins 1967), the development of liposomes for encapsulation (Gregoriadis and Ryman 1971; Gregoriadis et al. 1974), as adjuvants (Allison and Gregoriadis 1974), and targeting (Gregoriadis and Neerunjun 1975), and even early patents (Allison and Gregoriadis 1977). Gregory was also an enthusiastic and prolific reporter of each new advance in liposomes, bringing together the relatively small community of researchers in the then nascent field, through his series of review papers (Gregoriadis 1976) and edited books (Gregoriadis 1979), including the extremely influential three-volume Liposome Technology (Gregoriadis 1984), a series that still continues in its third edition (Gregoriadis 2010).
In his 1975 paper on homing liposomes, the abstract reads as follows:
The possibility of homing liposomes to target cells was investigated. Liposomes containing an antitumor drug and associated with molecular probes, which exhibit a specific affinity for the surface of a variety of normal and malignant cells, were prepared. In vitro and in vivo experiments suggested that such probes were capable of mediating selective cellular uptake of the associated liposomes and the entrapped drug. It is anticipated that liposomes designed to home may become important tools in the control of cell behavior.
This is 1975! This kind of “homing” work is still going on today in liposomes and, indeed, with a whole host of “nanoparticles.” Drug encapsulation and targeting is still being researched, and attempts are still being made to test clinically and eventually commercialize these approaches. Forty years later, it’s still a challenge. While I was trying to give advice from our own experiences with ThermoDox®, I suggest that these early papers still have something to teach us (especially the new generations entering the field of “nanomedicine”) about both research and the decision-making process during commercialization of all nanoparticles for drug delivery and imaging. So just because you and your advisor are choosing to work on nanoparticles of gold, iron oxide, chitosan, poly(lactic-co-glycolic acid), etc., and even antibodies, does not mean you should not look up, read, study, understand, and use this literature on liposomes to plan your studies and interpret your results.
23.1.2 EXAMPLE OF AN EARLY “LESSON LEARNED” IN LIPOSOMES: IS IT BEING HEEDED TODAY IN NANOMEDICINE?
For example, these early applications were hampered by the now relatively well-understood opsonization phenomenon that labels most nanoparticles in the bloodstream as foreign for removal by the reticuloendothelial system (RES) (Roerdink et al. 1981). In their early formulations (Gregoriadis et al. 1971, 1974), Gregoriadis and colleagues had used a composition of 40 μmol of phosphatidylcholine, 11.4 μmol of cholesterol, and 5.7 μmol of the negatively charged phosphatidic acid (molar ratio 7:2:1), giving liposome that was 10 mol% negatively charged. When Alec Bangham took me out to a Chinese dinner on the eve of my giving his 80th Birthday Lecture at Gregory’s 2001 5th International Conference on Liposome Advances, in London (Needham 2001a), he told me a story from those early days. Alec had explicitly advised that the initial strategies to make the liposomes “repulsive,” by including negative charge, which was thought to be electrostatically stabilizing, would conversely only result in liposomes going to the RES faster than ever. This has since been proven (Allen et al. 1988) and is fairly well understood (Liu et al. 1995) in serum and serum-free media (Rothkopf et al. 2005). As we now all appreciate, this opsonization to Kupffer cell removal of the liposome in the bloodstream was solved by applying earlier work on the PEGylation of proteins (Beauchamp et al. 1983), and the invention of the so-called “stealth” liposome (Allen 1989), with its sterically stabilizing polymer (Allen and Gabizon 1990; Klibanov et al. 1990), forming the basis for Doxil® (see Barenholz’s review and references therein, Barenholz 2012). There is also a lesser-known mechanism of a mechanical effect of high cholesterol content in PC lipids or sphingomyelin (Kim and Needham 2001; Needham and Nunn 1990), which gives a similar long circulation half-life (Lasic and Needham 1995), used to good effect (slow leakage and long circulation) in the vincristine liposome (Boman et al. 1993, 1994; Kanter et al. 1994; Krishna et al. 2001; Webb et al. 1995, 1996).
I mention these early studies and “lessons learned” because newbies (and “oldbies”) to the field of nanomedicine would do well to read through some of these early reports and seek to inform their own current research strategies. “Nanomedicine” does seem to know all about PEG-derived steric stabilization, but is it as well appreciated in the nanomedicine literature that a tight lipid-surface is also not well opsonized and that an amount of negative charge of only 3 mol% or greater (Allen et al. 1988) can send your precious nanoparticle rapidly to its RES fate?
As Frank Szoka (who entered the field in its “golden age”; Szoka and Papahadjopoulos 1978), famous for ranting in an editorial Commentary: Rantosomes and Ravosomes (Szoka 1998), reminded us, quoting the philosopher, essayist, and poet, George Santayana: “Those who cannot remember the past are condemned to repeat it.” The example Frank chose to illustrate this was liposomes carrying anti-HIV agents. He points out that
The difficulties with the approach could be anticipated based upon the early liposomal anti-cancer drug delivery literature. The failure of those working in the field is not because they repeated previously published studies. But rather because they did not translate the lessons from the early studies into a therapeutic strategy that complemented the pathophysiology of the disease, with the pharmacological aspects of the drug and the delivery properties of the liposome.
Thus, to reiterate in this editorial, Frank’s amendment to the Santayana quote was
Those who cannot translate the lessons of the past are condemned to repeat it.
Frank, correct me if I am wrong, but basically what I think you were saying is
Those of you who do not read the literature are condemned to repeat it! And guess what? We have already done it, and we are tired of hearing about your ‘new work’ at meetings, or seeing what you think is new and exciting in your grant proposals, that we have to take our precious time to read through, review, and reject.
Back then, the (liposome) world was certainly smaller, and as Szoka also points out, many of the potential uses of liposomes in drug delivery that have since come to be were discussed in those two 1976 review articles by Gregory Gregoriadis (1976). With only a few people involved, “discussions raged over materials, mechanisms, models, methods and structures” at the handful of liposome/drug carrier meetings that everyone attended.
Many lessons were, in fact, learned back then, face-to-face, between researchers during the meetings. But today, even with (and perhaps because of) easy access to the (voluminous) literature, this face-to-face contact is easily lost, and so the repetition of research and, indeed, the mistakes, still go on today. Frank, who rants on average about every 15 years, has another one. This time, it is directed at all nanomedicines and the people who research and develop them. Entitled “Cancer nanomedicines: So many papers and so few drugs!” (Venditto and Szoka 2013), folks would do well to heed it, as this most comprehensive review identifies a timeline to nanomedicine anticancer drug approval using the business model of inventors, innovators, and imitators. Unfortunately for today’s researchers who are still involved in “liposomes,” there is a lot of literature, some 48,772 publications currently listed (February 28, 2015) on a PubMed search for “liposome.” And incredibly, 339,246 patents that concern “liposome” at free patents online. “Lessons learned” from liposomes, like Chezy Barenholz’s landmark paper Doxil®—the first FDA-approved nanodrug (Barenholz 2012), will undoubtedly apply to all nanomedicines being researched and developed today. With only 9422 papers found on PubMed for a search of “nanomedicine,” it is likely that “your precious nanomedicine” shares many of the problems that were already figured out by the liposomologists, maybe before you were born or, at least, while you were still in grade school. So where would a company turn to, in order to get the most expert and up-to-date knowledge about their licensed product? Would they sift through the 48,772 papers and pay a law firm $gazillions to search through the 339,246 patents? No. Maybe try asking the inventor, and first see what he or she has to say (and more about that relationship later).
23.1.4 EARLY COMMERCIALIZATION AND ACCESS TO A WEALTH OF EXPERTISE: JUST ASK US
Early commercialization efforts were considerable (as well as contentious; Free Library 2014; NeXstar Pharmaceuticals 1997). From my perspective, there were four principal liposome companies: three were established in the United States: Liposome Technology Inc. (LTI) in Menlo Park, California; The Liposome Company Inc. in Princeton, New Jersey; NeXstar, based in San Dimas, California; and Inex in Vancouver, Canada, first as a spin-off from TLC and then in its own right. These were the main entities that, for me, paved the way in mainly anticancer liposomal therapeutics, took relatively similar drug delivery ideas, and brought them through the development and translation to commercialization. Two companies focused on liposomal doxorubicin, LTI (Doxil®) and The Liposome Company (EvacetTM and Myocet®), NeXstar went with daunorubicin (DaunoXome®) and also successfully developed amphotericin B (AmBisome®), and Inex developed liposomal vincristine (Marqibo®).
With academic researchers involved at varying levels of invention, innovation, and continued support, there is a whole host of very experienced individuals (still) around the conference, industrial, and grant-review world, whom students, postdocs, and young faculty new to the field can call on for advice and informal consultation. So, if you see Chezy Barenholz (Barenholz 2012), Frank Martin, or Martin Woodle et al. (Barenholz and Haran 1994; Papahadjopoulos and Skoza 1980; Woodle et al. 1989) ex-LTI-Sequus; Andy Janoff et al. (Janoff et al. 1989, 1996; Mayer et al. 1997) ex-TLC; Pieter Cullis, Lawrence Meyer, Marcel Bally, or Murray Webb et al. (Bally et al. 1997; Webb et al. 1996; Wheeler et al. 1999), ex-Inex; or Gary Fujii, Bill Ernst, Jill Adler-Moore, or Su-Min Chang et al. (Adler-Moore and Chiang 1997; Adler-Moore and Ernst 1997; Proffitt et al. 1999; Schmidt and Fujii 1998) ex-NeXstar; or very knowledgeable and outspoken independents, like Frank Szoka (Papahadjopoulos and Skoza 1980), Theresa Allen (Allen 1989; Allen and Gabizon 1990), or Valdimir Torchilin (Torchilin et al. 2006), or me and Dewhirst, at a meeting or seminar, we are all inventors of some of the earliest or later patents and a host of peer-reviewed papers that have contributed to those 48,772 publications. You might ask us one or more of the questions I am hoping to address in this chapter: What was the original observation that motivated the product? How did you or your colleagues have it? What was the question you tried to answer? What was the scientific hypothesis that you and your colleagues addressed? What funds were available for initial development and how did you get them? How did you develop it, including preclinical animal studies, and what were the results? What collaborators did you work with? What was the initial licensing deal and how was it structured and with whom? How did you manage the scale-up of manufacturing? What particular disease did you decide to treat and why? What did the Food and Drug Administration (FDA) require you to write in order to obtain an Investigational New Drug (IND) application? What funds did you have to raise, in order to carry out the IND application and how did you get them? How did you manage the Phase 1 trials, and what was the result? How did you recruit the clinicians and the sites to carry out the trials? Were there any unexpected problems implementing the protocols? Was that next stage a Phase 2 or did you go straight to Phase 3 human clinical trials? What was the next documentation you had to provide to the FDA and how long did it take to move to this next stage? What was the protocol? How many patients and sites did this Phase 3 involve, and how was it all managed? How did it turn out? Are you still in business? If so, what are your future plans for your drug delivery technology? In your view, what’s next? What’s the next big thing in advanced drug delivery and why? The answers are all available if you would just ask.
Invented in 1996 (Needham 2004), the LTSL is now in Phase 3 human clinical trials for liver cancer. This chapter will describe the engineering design of the LTSL (Needham 2013) and the licensing and clinical testing that is moving the invention toward commercialization. LTSLs, in conjunction with local mild hyperthermia (HT), can release drug within seconds of entering the warmed tumor vasculature. The released, free drug diffuses into the tumor interstitium, reaching its nucleus target with greater penetration distance, and to much higher concentrations, than those achievable by either free drug or the more traditional long-circulating liposome formulations (Manzoor et al. 2012). Intravascular drug release provides a mechanism to increase both the time that tumor cells are exposed to maximum drug levels and the penetration distance achievable by drug diffusion. This establishes a new paradigm in drug delivery: rapidly triggered drug release in the tumor bloodstream, saturating neoplastic cells, as well as endothelia, pericytes, and stroma, with the anticancer drug.
This chapter will attempt to describe the process, the pitfalls, the good, the bad, and the ugly of translating what might be a good research idea through initial funding, development, and preclinical and clinical trials. Whether unique or not (and we suspect the issues highlighted in our particular case are probably quite ubiquitous), I will use, as the main example, the invention, development, clinical testing, and intended commercialization of the thermal-sensitive liposome, subsequently named ThermoDox®. Additionally, an update of the recent progress in human clinical trials will be given, including Celsion’s Phase 3 for liver cancer, Phase 2 recurrent chest wall (RCW) cancer, and their new trials in metastatic liver cancer, ovarian cancer, pancreatic cancer, breast cancer, and glioblastoma, including the adaptation of high-frequency ultrasound (HiFu) as the source of targeted mild HT. I will say up front that the current Celsion administration should be commended for their pioneering work in taking on this invention, initiating this very impressive series of trials, with multiple sites, in different countries, and in particular for creating and carrying out what actually is the largest randomized double-blind trial ever with radio-frequency ablation (RFA). For example, following on from what was learned in the Phase 3 HEAT study on primary liver cancer, Celsion’s new OPTIMA trial is currently enrolling, with the first patient enrolled Q3-2014. It will include approximately 550 patients in up to 100 sites in North America, Europe, China, and the Asia-Pacific region.
Kudos to Celsion and in particular Mike Tardugno (CEO) and Nick Borys (CMO); it’s not an easy task visiting all these clinical sites and spending multiple days on airplanes.
Following the licensing of my (Oops, sorry, no, it’s not mine!) Duke’s invention by Celsion Corporation in November 1999, ThermoDox® has now, just over 15 years later, completed a Phase 3 trial in 701 patients with primary liver cancer, where ThermoDox® + RFA was trialed against RFA alone (Poon and Borys 2009; Wood et al. 2012). There were certainly lessons learned in the science (and all that is published in several reviews; Landon et al. 2011; Needham 2013; Needham and Dewhirst 2001, 2012) and the seminal paper on drug accumulation and penetration by Manzoor et al. (2012). But why we think there are real lessons to be learned in the development and commercialization from all this in particular, is the fact that when the 701-patient Phase 3 primary liver cancer trial results were unwrapped by Mike Tardugno and colleagues, on January 31, 2012 (Tardugno 2013), the headline read as follows:
ThermoDox® failed to meet its primary endpoint of better progression free survival than the heating modality (RFA) alone.
As described later, data are still being analyzed and some encouraging results are being revealed, and further trials are being conducted including new heating modalities like HiFu. A lot of lessons have been learned (especially) by the inventor and also by his collaborators, the university licensing and ventures office, the faculty patent committee, and the company and the clinicians, which will still bring this particular treatment option to oncologists, their patients, and the clinic. Taken largely in chronological order, this chapter will detail the events and lessons learned and address many of the questions listed in Section 23.1.4 so that others, with potentially good ideas, compelling in vitro, and in vivo data, can perhaps have a smoother path to testing, and, if successful, commercialization of their own idea.
The dedicated Section 23.7 on “Lessons Learned” will present six main topics:
• Your university administration
• Your Office of Licensing and Ventures (OLV)
• Your license agreement
• Meeting the milestones
• Your (inventor’s) relationship with the company
• The distribution company
I hope this will form a basis for discussion, if not, identify pitfalls that others might avoid, or at least be aware of, and some of the reasons they could occur. There will also be a few lessons as we go, in dedicated boxes, just for good measure.
The chapter will end with a brief description of an approach we are currently pursuing to formulate especially hydrophobic anticancer drugs specifically to metastatic disease. This approach has again benefited from lessons learned in the liposome and other nanomedicine fields. It is an endogenous one, which is based on the way nature delivers her own hydrophobic molecules in the body, what we call “Put the Drug in the Cancer’s Food.” And it gives me one last chance to make a difference. With my group here in Odense, Denmark, I want to do it in such a way that it is not encumbered by the kinds of events that have hampered ThermoDox® and other products. I therefore introduce what might be a surprising concept, translating drug delivery without profit and open-source pharmaceuticals, especially for cancer. Whether this approach might help to eliminate the negatives that sometimes cloud these translational issues of hidden agendas, incompetence, and greed and replace them with transparency and the right expertise for the right job, and an opportunity to provide healthcare to the people, which is not encumbered by the promise of huge profits, is still to be determined. This may not be possible in some countries, but the more socially minded ones, perhaps in Europe, indeed like Denmark, the “cancer capital of the world” (WCRF International 2015), could maybe lead the way in this new thinking about translating drug delivery and making our research advances actually available to the people who suffer, at cost.
The story proper starts with the initial motivation by Dr. Mark Dewhirst, the then director of the Duke Hyperthermia Program, who, in about 1995, quite explicitly said “Liposomes aren’t working, Hyperthermia isn’t working, I need something I can heat and it releases drug, damn it.” It was Mark then, who motivated the need to come up with a possible solution: a fast drug-releasing thermal-sensitive liposome, disclosed to Duke in 1996 (Box 23.1).
As shown in Figure 23.1, in 1995, three timelines intersected:
1. Dox was discovered and was one of the first choices of drug for encapsulation in some of the first liposomes to be made for testing against cancer.
2. HT as a treatment modality had been developed and was being used to heat tumors.
3. Lipid membrane mechanochemistry used micropipette manipulation techniques to measure the properties of single-walled lipid vesicles.
BOX 23.1 LESSON AS WE GO #1: LISTEN TO YOUR CUSTOMER
And here’s the first lesson. Listen to the people on the front lines, the clinicians, the veterinarians, and the directors of centers, and see what “your customer” might actually want. As I discuss at the end of this chapter, the new formulation we are now working on was requested, or at least motivated, by a need expressed by several actual medical oncologists, asking, “can you help us to reformulate lapatinib, niclosamide, fulvestrant, abiraterone, and orlistat?” So does your formulation fulfill an actual clinical need? If not, consider not doing it (at least not just for the papers) and challenge your PhD or postdoc advisor and ask them: Does what you want me to do pass the “so what test”? Is it useful knowledge? Does it even attempt to meet and unmet need? If you are not sure, you probably know the answer, but you still might want to canvas some end users over at your local cancer center.
FIGURE 23.1 In 1995, three timelines intersected. Doxorubicin and its encapsulation in liposomes, hyperthermia used to heat tumors, and lipid membrane mechanochemistry, using micropipette manipulation techniques to measure the properties of single-walled lipid vesicles.
23.2.1 DOXORUBICIN AND LIPOSOMES
When we decided to develop the LTSL technology, we simply inherited the “stealth” liposome concept and the cytotoxic drug, Dox, which had already been used in liposomal delivery. And so, as a prelude to discussing actual ThermoDox®, it is worth a relatively brief (not exhaustive) review of what we knew about liposomes and Dox in 1995, highlighting a few comparisons, even a few lessons learned as we go, and exactly what it was about liposome technology and its performance at the time that motivated the LTSL invention and its development.
23.2.1.1 Doxorubicin, the Drug
Dox (also known as hydroxydaunorubicin and hydroxydaunomycin; trade name, Adriamycin®) is an anthracycline antibiotic discovered in the early 1960s by the Farmitalia Research Laboratories of Milan. Clinical trials began in the 1960s, and the drug saw success in treating acute leukemia and lymphoma. It was approved for use in the United States in 1974. However, by 1967, it was already being recognized that Dox (and another anthracycline chemotherapeutic, daunorubicin), while active against cancer, could produce fatal cardiac toxicity.
FIGURE 23.2 Doxorubicin (Adriamycin). (a) Molecular composition and structure. (b) Diagram of two doxorubicin molecules intercalating DNA.
The structure and mechanism of action of Dox is shown in Figure 23.2. It is known to intercalate between DNA base pairs (Frederick et al. 1990), resulting in DNA and DNA-dependent RNA synthesis inhibition due to template disordering and steric obstruction (Abraham et al. 2005). By virtue of this ability to intercalate with DNA, it stabilizes the topoisomerase II complex after it has broken the DNA chain for replication, preventing DNA double helix from being resealed, stopping the process of replication (Frederick et al. 1990).
Dox has one of the widest spectrums of any neoplastic agent and is commonly used to treat Hodgkin’s lymphoma and some leukemias, as well as cancers of the bladder, breast, stomach, lung, ovaries, thyroid, soft tissue sarcoma, multiple myeloma, and others. Toxicities exhibited include myelosuppression, alopecia, mucositis, nausea, vomiting, and cardiomyopathy. Due to the cardiotoxicity being additive, the cumulative lifetime dose is limited to ≈550 mg/m2. The cardiotoxicity is most likely a consequence of free radical generation and binding of the drug to cardiolipin in the heart muscle.
Dox also interacts with the cell’s electron transport chain, to lead to the formation of superoxide anion radicals and hydrogen peroxide, which has a very damaging effect on the cellular components. Because of its anticancer activity and also because of its intense toxicity, its encapsulation in liposomes was one of the biggest early successes. Liposomal encapsulation reduced its toxicity. The flip side of reduced toxicity though could be reduced efficacy, and subsequent clinical data support this formulation conflict (O’Brien et al. 2004), where in first-line therapy for Metastatic Breast Cancer, PEGylated liposomal Dox (PLD) significantly reduced cardiotoxicity, myelosuppression, vomiting, and alopecia but only provided comparable efficacy to free Dox. Still, with the widespread prescribing of Dox, as O’Brien et al. suggest, for elderly patients and patients with specific cardiac risk factors, PLD was an important new therapeutic option.
As is well known, after intravenous (i.v.) dosing, Dox blood levels fall dramatically as the drug distributes into the tissues, followed by a slow elimination phase due to renal and biliary clearance and metabolism. Hence, worth reproducing, and as shown in Figure 23.3, Dox itself has a half-life of only ≈1 minute (Gustafson et al. 2002). But, as a chemical, Dox has some very interesting physicochemical properties. With a pKa of 8.3, it is a weak base cation, such that at pH 7.2, ≈10% of it is uncharged and 90% of it is positively charged. What this means is that the uncharged fraction of Dox can pass through the whole tissue, simply by concentration-dependent, Fickian diffusion. Permeation (and thus, loss) of the neutral form through the membranes drives the equilibrium disassociation reaction forward, releasing more uncharged fraction in the interstitial fluid, which is then available for further transit through each cell membrane. Uncharged Dox also partitions into the cytoplasmic and organelle membranes; it passes through and may have a direct, or indirect, toxicity effect there too. In fact, following Dox treatment, cardiotoxicity develops through the preferential accumulation of iron inside the mitochondria, due to Dox becoming concentrated in the membranerich mitochondria and increasing both mitochondrial iron and cellular reactive oxygen species levels (Ichikawa et al. 2014). Also, because of this dual solubility afforded to such weak base cations, once partitioned in, it takes a long time to get back out. It could be lethal in so many ways, if only we could get the drug just to the tumor vasculature, and in high quantities.
23.2.1.2 Doxorubicin Encapsulated in Liposomes
Dox was already approved and formulated in a non-PEGylated liposomal form (Myocet®, formerly known as Evacet™) (Abraham et al. 2005) but, as predicted by Bangham from the outset, “traditional” liposomal formulations showed too rapid a circulatory clearance and little efficacy. This was followed by Doxil® (marketed as Caelyx® in Europe), the PEGylated “stealth”* liposome (reviewed comprehensively in the edited book Stealth Liposome by Lasic and Martin, 1995; also Lasic and Needham, 1995 and also described in Chapter 5, Section 5.5.1.2). Although it was later than the traditional liposomes in its development, Doxil® was the first liposomal pharmaceutical product to receive U.S. FDA approval in 1995, for the treatment of chemotherapy refractory, acquired immunodeficiency syndrome–related Kaposi’s sarcoma. Thus, as we were thinking about how to solve the problem that Mark Dewhirst identified, the field was buzzing with news of FDA approval for Doxil®. Doxil® certainly played a huge part in getting this liposomal technology into mainstream use, and as reviewed recently by Chang (Chang and Yeh 2012), as of 2012, there were 12 liposome-based drugs approved for clinical use and more are in various stages of clinical trials.
The success of Doxil® and its stealth, PEGylated polymer design was to escape RES uptake and create a long-circulating liposome that could stand a chance of extravasating into the tumor interstitium via the purported enhanced permeability and retention (EPR) effect. We had even already shown this ourselves in 1993 (Wu et al. 1993). Figure 23.4 shows the intravital microscopy images after fluorescently labeled stealth liposomes were injected i.v. into rats bearing Dewhirst’s dorsal skin-flap window chambers, containing a vascularized mammary adenocarcinoma.
The bright-field image in Figure 23.4a shows the complex and resurgent flow of the tumor vasculature, where, for scale, the larger blood vessels are about 30 μm in diameter. Figure 23.4b is taken just 1 minute after i.v. injection of the 82 ± 24 nm diameter stealth liposomes into the rat’s tail vein (Wu et al. 1993). Switching the microscope to epifluorescence illumination, the liposomes are clearly flowing in the blood vessels and, even at this early time point, have started to extravasate into the tumor interstitium. Ninety minutes later, not yet having set our optimal low-light-level camera settings, we had to turn the gain on the fluorescent camera down to get the last image in Figure 23.4c, where there was a significant, but heterogeneous, accumulation in the tumor perivascular space.
As shown in Figure 23.5, when the data were quantitatively analyzed, the longer circulation halflife correlated with there being more stealth liposome accumulation in the tumor interstitium than for the more conventional non-PEGylated (91 ± 41 nm diameter) liposomes.
And with all this actual in vivo data, we were part of helping Doxil® to get approved, by showing that the extravasation actually happened in real time within 90 minutes or less. In fact, normal vasculature showed no extravasation at all. The importance of these data then was that it seemingly supported the Doxil® data from Vaage and Mayhew (Vaage et al. 1992) that had prompted our window chamber study in the first place. The EPR effect was alive and well (in this subcutaneous animal model). The passive accumulation of long-circulating stealth liposomes was an unqualified success (again, it must be emphasized “in this subcutaneous animal model”).
So, why would anyone need to improve on this?
It didn’t take long for data to start accumulating in the literature that even though there was now a long enough time to allow for passive extravasation, there were other challenges:
• Stealth liposomes might not be able extravasate to the extent that we had been seeing in subcutaneous animal tumor models.
• If they did, “this is as far as your liposomes can go,” i.e., only as far as the perivascular space.
• As Yuan et al. in 1994 had shown, using a similar window chamber technique (Yuan et al. 1994), the intramural accumulation of liposomal fluorescent spots (observed within 5 minutes after liposome injection) could be continuously observed for up to 2 weeks.
• No one knew exactly how leaky a human tumor vasculature really was to these ≈100 nm liposomes.
• No one was really sure to what extent, and at what rate, the liposomes, which had been well optimized for the best encapsulation of Dox, could even release their drug in the tumor interstitium.
• In very recent studies that have sought to provide a mechanism whereby Doxil® could potentially leak its drug, in an environment of ammonia (Silveram amd Barenholz 2015), it was found that PLD without ammonia had a “very poor cytotoxicity,” demonstrating again that Doxil® does a very good job of retaining its drug, but is not designed to release it.
So, quietly, between ourselves, Mark Dewhirst and I had questions in 1993–1994, born out of our own preclinical animal studies: “could Doxil® really deliver enough drug to human tumors to achieve significant efficacy?”
Attempting to improve Doxil’s efficacy by using HT to increase the vascular permeability, the Hyperthermia Center at Duke also carried out two “Doxil® + HT” clinical trials in ovarian and breast cancers (Secord et al. 2005; Vujaskovic et al. 2010). Unfortunately, the clinical trial evaluating the combination of Doxil® + HT in patients with persistent and recurrent ovarian cancer did not demonstrate increased efficacy, compared with prior reports using Doxil® alone. And in a Phase 1/2 study of paclitaxel in patients with locally advanced breast cancer in the preoperative setting, the pathological complete response (pCR) rate was only 9% for liposomal Dox (Evacet™) + local HT, although the combined (i.e., paclitaxel + liposomal Dox [Evacet™] + local HT) pCR rate was 61%.
Although we did not know it at the time, therapeutically all these concerns were, in fact, borne out in subsequent human trials, including a trial that was instrumental in gaining Doxil’s approval for ovarian cancer. A trial for metastatic breast cancer showed that Doxil® really was no better than free drug alone (O’Brien et al. 2004). That is, even though Dox has a half-life of only 2 minutes and Doxil® has a half-life of 73.9 hours:
• The overall survival was comparable with both treatments.
• Median: PEGylated liposomal Dox 21 months, versus doxorubicin, 22 months; hazard ratio (HR) = 0.94 (95% CI 0.74–1.19).
• At the time of the analysis, approximately 56% of patients in each group had died. When adjusted for potential imbalances in prognostic variables using the Cox regression analysis, the HR was 0.94 (95% CI 0.75–1.19), similar to the unadjusted treatment HR.
Doxil® was approved for ovarian cancer (Gordon et al. 2001) in 2005, but again, with little therapeutic benefit, when compared to topotecan:
• Time to progression (TTP): Doxil®, 4.1 months, and topotecan, 4.2 months
• Overall median survival: Doxil®, 14.4 months, and topotecan, 13.7 months
• 18% reduction in risk of death (hazard ratio [HR] = 1.216)
• Overall tumor response rates: 19.7% (47 patients) in the Doxil® arm and 17% (40 patients) in the topotecan arm
Compared to topotecan then, improvement in TTP for Doxil® was 0.1 month, which is only a 2.4% progression-free survival (PFS), and it was approved. TTP was also a measure for ThermoDox®, and we will see later how it faired (when taken in total, it didn’t fare well), in relation to the FDA-required %PFS stipulated for its approval.
23.2.1.3 New Toxicities for Doxil®
As mentioned earlier, the initial success of non-PLD (Myocet®) was to reduce the drug’s cardiotoxicity, by simply encapsulating and retaining it; Doxil® certainly achieved the same retention and reduced cardiotoxicity profile. However, the long circulation half-life also introduced new toxicities: hand–foot syndrome or palmar–plantar erythrodysesthesia (PPE). PPE is a common dermatologic toxic reaction associated with certain chemotherapeutic agents, including continuous infusion Dox, cytarabine, floxuridine, high-dose interleukin-2, docetaxel, capecitabine, vinorelbine, and gemcitabine. It presents as a redness, swelling, and pain on the palms of the hands and/or the soles of the feet; sometimes blisters appear. In a study in 2007 by Lorusso et al. (2007), the incidence of PPE is increased in patients receiving PLD compared with conventional Dox. In studies that utilized the currently approved dose of PLD (50 mg/m2 every 4 weeks), ≈50% of all patients receiving PLD experienced PPE and ≈20% experienced grade 3 PPE. It has been hypothesized that following local trauma associated with routine activities, PLD may extravasate from the deeper microcapillaries in the hands and feet. Interestingly then, that passive accumulation in tumors—which could help in its therapeutic effect—may be compromised because of limited extravasation, whereas a similar extravasation of PLD in active hands and feet introduces a new toxicity (Box 23.2).
Given these successes of the Doxil® formulation:
• Good encapsulation and retention of a toxic chemotherapeutic during the blood-borne delivery phase
• A reduction in the cardiotoxicity for the drug
• Long circulation half-life that at least gives the chance of EPR
but coupled with its discovered limitations of:
• Low EPR in human tumors
• Introduction of known drug-associated toxicities for the liposome formulation
BOX 23.2 LESSON AS WE GO #2: DESIGN, DESIGN, DESIGN
Do as much forward engineering design for your formulation as possible before and during the research and development phase. A function that has been designed for therapy can often lead to a second negative performance characteristic. In fact, I would encourage you to follow the design methodology I laid out in a review chapter for a different Kinam Park–edited book: “Reverse Engineer the Low Temperature Sensitive Liposome (LTSL)” (Needham 2013). In it, I take the LTSL as an example and outline a design process that not only describes all aspects of the liposome and how it is used, but also provides a general scheme that, when followed for any device or product or “problem that has already been solved,” allows a person to formally reverse engineer that product. In doing so, the process itself becomes an invention generator for material improvement or inspiration for new designs and, when used in a forward engineering sense, is the template for your new research and development effort. As discussed further in Section 23.8, we did that for the LDL project we are pursuing now in Denmark, and this process ensures a more knowledgeable design.
• Limited penetration of the 80 nm diameter liposome into the tumor interstitium
• Lack of effective delivery to all tumor cells (due to the same good retention of the drug)
I felt that in trying to respond to Mark’s need of “something I can heat and it releases drug,” the main challenge was to try to keep the good parts, i.e., the retention of Dox in the liposome while in the bloodstream (although this was somewhat compromised, as we will see later), but to also get the drug out, fast, and only at the tumor. Since there was a well-established maximum tolerated dose (MTD), the challenge was actually less about reducing toxicity and more about increased bioavailability in delivery, thereby gaining actual efficacy.
While all this liposomal development was going on, we also knew that tumors could be heated using HT, or at least Mark Dewhirst did. Mark and his colleagues had been working in HT since the early 1980s. In a monograph from 1983 (Oleson and Dewhirst 1983), Oleson and Dewhirst focused on progress at the time regarding “major aspects of the biologic effects of elevated temperatures both in vitro and in vivo, on the physical methods clinically used to produce HT, and on the results of treatment in large animals and humans.” It is well recognized that HT can have at least three different effects on cells:
• Direct cytotoxicity at elevated temperatures, where the degree of cell killing is both time and temperature dependent
• Heat sensitization of radiation, where HT reduces cancer cells ability to repair sublethal and potentially lethal radiation damage
• Synergistic effects with certain drugs, including alkylating agents, nitrosoureas, cisplatinum, bleomycin, and adriamycin, which showed marked synergism above 43°C
There were also distinctions between the temperatures attained and the desired effect. Mild HT is a therapeutic technique in which cancerous tissue is heated above the body temperature to induce a physiological or biological effect but often not intended to directly produce substantial cell death. The goal is to obtain temperatures of 40°C–45°C for time periods up to 1 hour (Issels et al. 2010; Viglianti et al. 2010). In contrast, ablative HT is commonly greater than 55°C, but for shorter durations of 20 seconds to 15 minutes (Wood et al. 2002). An example would be the relatively recent, HiFu (Kennedy 2005), and the older and more traditional, RFA, in treating lesions in primary liver cancer (Tateishi et al. 2005). RFA is used in conjunction with imaging techniques (e.g., ultrasound, computed tomography, or magnetic resonance imaging) to help guide a needle electrode into a cancerous tumor. High-frequency electrical currents are then passed through the electrode, creating heat that destroys the abnormal cells. An area of ≈30 cm2 (≈3 cm diameter tumors) can be ablated with a single application of RFA (Goldberg et al. 1996). While HiFu is a technique that Celsion is now planning to use, RFA was the heating modality Celsion chose to use in the human clinical trials, in order to raise the temperature of the one or more liver lesions that would be exposed to ThermoDox® (more on this later in Section 23.6).
Given that there are at least 25 different sites for cancers, the ability to heat and provide HT is only limited by the engineer’s ability to design and build an applicator, catheter, or focused radio-frequency (RF) array, to provide the heat. Thus, most parts of the body can be heated by HT using especially designed applicators for breast and head and neck cancer, a focused RF array for abdominal tumors, a catheter for prostate cancer, a microwave cap for brain cancers, and an RFA probe for liver cancer.
As will be discussed later, while RFA is a widely used technique and certainly has the capacity to burn away the center of the tumor, Celsion’s plan was to target the micrometastases at its edge, thought to be responsible for disease recurrence, with ThermoDox®. However, the control over the temperatures attained at the tumor margins was not well characterized, and so this became a “heat-and-hope” strategy. Also, the continual heating that we had applied using a simple “leg-in-the-water-bath” technique in the mouse studies was not possible here. RFA is applied full-on for 6 minutes, and additional heating can only be given in a cycled fashion.
In contrast, Celsion had already fully characterized their Prolieve® Thermodilatation System (Prolieve®) technology for benign prostatic hyperplasia (BPH). This catheter-based system was approved for use in BPH in February 2004 as being capable of heating the whole prostate. Prolieve® has undertaken a Phase 4 postmarketing study to evaluate the long-term safety and effectiveness in the treatment of BPH (Weiner 2015). So with an FDA-approved treatment, Celsion started a Phase 1 study with William Gannon as the CMO, which could easily have provided good solid data on temperature and drug release and even resulted in a chance at approval for ThermoDox®. So, what went wrong here? Despite encouraging efficacy data in Phase 1, Celsion decided against finishing it for “business reasons,” which are described further in Section 23.6.1.
The bottom line for HT though, in the context of the LTSL, is that now we had a drug–device combination, with several devices that could effectively heat many different parts of the body, where tumors might grow. If the engineers could heat it, LTSL would release its drug, which was the underlying tumor-targeting strategy.
23.2.2.1 Effects of Hyperthermia
As shown in Figure 23.6, HT has some effects alone (Dewhirst and Sim 1984; Oleson and Dewhirst 1983), is synergistic with drug (Storm 1989), increases blood flow and vascular permeability to macromolecules (Wu et al. 1993), and enhances liposome extravasation (Kong and Dewhirst 1999; Kong et al. 2000, 2001; Li et al. 2013; Matteucci et al. 2000). But the new concept (Needham 1999, 2001b, 2004) was HT-induced rapid triggered release of drug from the LTSL (Anyarambhatla and Needham 1999; Mills and Needham 2005; Needham 2001b, 2013; Needham and Dewhirst 2012; Needham et al. 2012; Wright 2006) that was only initiated in the bloodstream of the tumor (Needham and Ponce 2006), resulting in deeper penetration of Dox to all cell nuclei throughout the tumor interstitium (Landon et al. 2011; Manzoor et al. 2012), leading to an increased therapeutic effect in in vivo preclinical models (Kong et al. 2000; Needham 2001b; Yarmolenko et al. 2010) and in canine (Hauck et al. 2006) and human clinical trials (Poon and Borys 2009; Vujaskovic 2007; Zagar et al. 2014).
So what was the underlying science? What were the observations and questions that generated the invention? It was 15 years of lipid membrane mechanochemistry.
23.2.3 LIPID MEMBRANE MECHANOCHEMISTRY
Following the pioneering characterization of the red blood cell (RBC) membrane in the 1970s (Evans and Hochmuth 1976), “lipid membrane mechanochemistry” as a field of study for pure lipid bilayers was really initiated in the early 1980s by Evan Evans, when he moved in 1980 from Duke Biomedical Engineering to the University of British Columbia, Vancouver, Canada. But he started endogenously, by checking out nature’s own designs for lipid-based capsules.
23.2.3.1 Starting with Red Blood Cells
While at Duke (1973–1981), Evans had developed and used the micropipette manipulation technique to characterize the mechanical properties of RBCs. Interestingly, the biochemists (Steck 1974) were only just starting to identify the composition and organization of the spectrin membrane cytoskeleton (Bennett 1982) at the same time that Evan was measuring with the micropipette technique (Evans 1973a,b; Evans et al. 1976). Thus, sophisticated mechanical models of the RBC membrane were introduced in the early 1970s by both Skalak (Skalak et al. 1973) and Evans (Evans 1973a). Evans unified a new material concept for the RBC membrane, which provided the capability of large deformations exhibited by normal discocytes as a two-dimensional, incompressible material, and a general stress–strain law was developed for finite deformations (Evans 1973). What followed was a period of intense activity (Evans and Hochmuth 1977, 1978), culminating in the seminal book by Evans and Skalak Mechanics and Thermodynamics of Biomembranes (Evans and Skalak 1980). Thus, for over 50 years, these micropipette techniques have provided the unique ability to apply well-defined stresses for dilation, shear, and bending modes of membrane and cellular deformation. They laid the foundation for similar micromechanical experiments applying well-defined stresses to single-giant unilamellar vesicles (GUVs) that, in turn, eventually generated the “idea” for the LTSL. This is how that happened.
23.2.3.2 Giant Unilamellar Vesicle Experiments
The classic materials engineering approach to either understanding an existing design (like the red or white blood cell) or creating a new design for a nanoparticle drug delivery system like a liposome is the same. It involves as complete an understanding as possible for the composition–structure–property (CSP) relationships of the materials involved in the component design. To be fair, most liposomologists did not have access to the more mechanochemical techniques we were using, and so in contrast to the development of most liposome and nanomedicine formulations, we didn’t just come up with an all-encompassing series of lipid–drug compositions and evaluate them for performance; we came up with one. Of course, this was based on liposomes that had already been developed and tested, especially the stealth version (Allen 1989). But with over 20,000 papers in the literature on liposomes at the time, new innovation can often require a deeper understanding of CSP relationships. And so, by the time Mark Dewhirst asked for a formulation he could “heat and it released drug,” we (and Evans before me) had been studying membrane materials science for over two decades and had already evaluated many of their CSP relationships using the micropipette technique. These micromechanical methods and the resulting data on single-bilayer vesicles have allowed us to characterize the lipid bilayer membrane in both a liquid and a solid state, including the influence of cholesterol on membrane elasticity and tensile strength. It was these kinds of direct measurements of the “two-molecule-thin” material that were, literally, instrumental in helping to characterize liposomal systems and explain much of the processing and performance of the liposome.
Starting in the 1980s, the micropipette technique was adapted and developed by Evans and Kwok (Evans and Kwok 1982; Kowk and Evans 1981) and then established by (Evans and Needham 1987), to study individual GUVs of various lipid compositions. A particularly historical and newly insightful perspective on the mechanics and thermodynamics of lipid biomembranes has been given recently (Evans et al. 2013), which emphasizes “the inherent softness of fluid–lipid biomembranes and the important entropic restrictions that play major roles in the elastic properties of vesicle bilayers.” In particular, the properties of importance for the LTSL were
• The membrane elastic modulus and other mechanical properties that determine drug loading and retention
• The nature of its main acyl-melting phase transition, which was the key trigger for drug release
• The behavior of the gel-phase membrane in shear and in particular its degree of yield shear and shear viscosity, as a result of in-plane shear deformations of membrane-grain structure
• Molecular exchange with water-soluble and membrane-soluble molecules like lysolipids that modified the membrane to allow it to have such a rapid release of encapsulated drug
• The adhesive and repulsive interactions that help maintain stability in blood circulation
Tables 23.1 through 23.5 show each of the most important and influential micropipette experiments that directly led to the LTSL invention. These key experiments will now be briefly described, along with the video images of the micropipette and GUV on which the experiments were carried out and their typical results. Students, please take note of the depth of inquiry, understanding, and especially mechanism, which such measurements and analyses have revealed. When I say you have to understand as much as you can about structure–property relationships of your nanomedicine material, in order to put mechanism between composition and performance, this is what I am talking about.
The first experiment and analysis that was carried out on single-walled GUVs by micropipette was to measure the elastic expansion and failure of egg lecithin bilayers (Kwok and Evans 1981). Summarized in Table 23.1, this experiment was similar to what had been used to measure the elastic modulus of RBC membranes (Evans et al. 1976)—a favorite inspiration for liposomes. It was used again by Needham and Nunn to characterize SOPC* and SOPC/cholesterol systems (Needham and Nunn 1990) and was further refined to include the contribution of membrane undulations by Evans (Evans et al. 2013), again, as a function of lipid composition including cholesterol content. For comparison, typical monounsaturated dichain lipids such as SOPC form lipid bilayers that are relatively soft (KA = 200 mN/m), weak (Tlys = 6 mN/m), and somewhat permeable to water and other materials (Bloom et al. 1991). The inclusion of 50 mol% cholesterol in bilayers composed of saturated chain lipids like DSPC, or hydrogenated soy lecithin (again a favorite compositional strategy for making liposomes), have KA ≈ 2000 mN/m, being 10× as stiff and 10× as strong, and relatively impermeable to water (Bloom et al. 1991). In their review of the Stealth Liposome, Lasic and Needham correlated such micromechanical lipid membrane data with liposome circulation half-life, showing that it is not just PEG and steric stability that can underlie extended half-life of a liposome in the bloodstream (Lasic and Needham 1995). The tighter the interface, the longer the liposomes could circulate. This explains the successful “stealth” effect of Cullis et al.’s (non-PEGylated) sphingomyelin–cholesterol–vincristine liposomes, with a measured elastic modulus of ≈2000 mN/m (Needham and Nunn 1990). These data helped us understand the role of mechanics in liposome-behavior: how it retains the drug and aspects of membrane permeability to water (at least), and how it evades the body’s defenses, with both steric, and mechanical, mechanisms.
TABLE 23.1Elastic Expansion and Failure of Membranes
By osmotically shrinking a single vesicle by just a few percent, thereby creating sufficient excess membrane area (compared to a sphere of the same volume) and supporting this membrane under low tension in the micropipette, I was able to take a single-vesicle membrane through its complete liquid-to-solid main acyl phase transition, Tm. For the SOPC/POPC-mixed system shown in Table 23.2, by measuring the area changes of the single vesicle, we could provide a phase diagram for this lipid mixture as shown in the plot. In an earlier study (actually my first ever study using the micropipette system and GUVs with Evans) reported in a very comprehensive paper on the thermomechanics of DMPC vesicle membranes (Needham et al. 1988), we showed how the liquid membrane underwent a tension-free transition into the L phase of over 30% change in lipid membrane area, consistent with the corresponding single lipid molecular area change from DMPC from 64 to 42 Å2. We also showed how the membrane entered the known Pβ′-rippled phase with tilted acyl chains, measured the mechanics of this rippled deformation, and, from the slopes of the area vs temperate plots, obtained the thermal area expansion coefficients in each liquid (Lα), semisolid (Pβ′), and solid (Lβ) phases. It was interesting to note that the changes in relative area per molecule at Tm and the pretransition Tp of 22% and 4% were in a similar ratio to the respective excess-specific heats reported from differential scanning calorimetry (DSC) measurements (Lentz et al. 1978; Mabrey and Sturtevant 1976), i.e., in the ratio 5:l, thus showing how our single-vesicle mechanocalorimetry agreed with traditional DSC. We also studied the influence of cholesterol in broadening and eventually obliterating any main acyl chain–melting transition, using again DMPC GUVs (Needham et al. 1988).
TABLE 23.2Main Acyl Solid–Liquid Phase Transition Video Micrographs
During this DMPC phase transition work (Needham et al. 1988), unpublished observations showed that a single, tension-free vesicle below the phase transition was faceted in appearance. Additional evidence for the presence of grains was actually seen in this transition experiment. If you look closely at the magnified insert of the solid lipid–melting image (Table 23.2b circled for your attention), there is a slight “kink,” which represents a grain boundary. Holding the vesicle with just a slight supporting suction pressure, as the temperature was raised and the lipid vesicle melted, its area per molecule expanded, the total area of the vesicle expanded, and it reversibly returned to its original melted liquid area. The (even more) interesting event was that, as it expanded, it clearly had to go through the mixed phase region of solid plus liquid domains, and we actually saw a lumpy-bumpy motion of the outer vesicle portion as “icebergs” of still frozen lipid in the melting bilayer moved past the glass pipette tip. Thus, for this SOPC/POPC lipid mixture, we showed that a vesicle became solid below its main acyl transition. But the next question was, “How solid?” In fact, the more mechanically oriented question was, “Is it a solid-like material (with elasticity), or does it have liquid-like character (and flows)?” It turns out it has both, behaving like a classic Bingham plastic with an initial elastic region, a plastic yield, and a viscous flow when deformed in in-plan shear. Next then is this experiment and the result that tested and measured this property of gel-phase bilayers, a state our LTSL would eventually be in, when injected into the bloodstream and then warmed through this transition by the local HT; whether in the blood stream or in a test tube, a property is a property.
By considering the gel-phase vesicle again with excess membrane area (over a sphere of the same volume), deformed into a micropipette, Evans came up with a membrane-mechanical analysis of the shear deformation of this single gel-phase vesicle that would measure the yield shear and shear viscosity of such DMPC membranes (Evans and Needham 1987). Then, as usual, it was my job to do the experiment and make it represent exactly the boundary conditions and assumptions in the theory. Table 23.3 shows this experiment for a smoothed out DMPC vesicle below its main acyl chain phase transition at a temperature of 13°C* (Needham et al. 1988).
In the experiment, a gel-phase vesicle is aspirated into the micropipette and formed into an outer spherical portion with a long projection into the pipette itself (as shown in Table 23.3d). I then release the pressure and gently blow out the vesicle, turn it around, and reaspirate it, axially with the projection. In applying a small suction pressure, the idea is to then find the point at which the elastic shear deformation of the membrane is exceeded, i.e., its elastic limit (Table 23.3a) at its plastic yield. This occurs when the projection in the pipette just reaches one pipette radius (1 Rp). Below this point, the material would return elastically to its original shape; beyond this plastic yield, the membrane would flow in shear. And so, a suction pressure of six times this yield pressure is applied and the vesicle duly flows into the pipette (Table 23.3b and c), with plug flow at the projections and positive and negative shear near the mouth and at the back end, respectively, until it is completely reaspirated (Table 23.3d). This behavior, of an elastic yield and viscous flow, is similar to more traditional materials that are termed “Bingham plastics” (Bingham 1922). In our highly cited 1987 review paper† (Evans and Needham 1987), we suggested that the shear rigidity and shear viscosity primarily reflected the density and mobility of crystal defects in the membrane bilayer surface. Thus, even a two-molecule-thick bilayer in its solid Lβ gel phase can deform with in-plane shear by grain sliding at intergrain boundaries (Kim et al. 2003). Ole Mouritsen and Martin Zuckermann (1987) had theorized that grains existed for single-phase membranes, in their analysis of the phase transitions for another similar PC lipid, DPPC, and we will see where this fits in later, when I discuss mechanisms of drug release at the phase transition of the LTSL. In fact, grain structure is clearly evident in the LTSL itself, as in the lower panel showing a transmission electron micrograph (TEM) of a Dox-loaded LTSL of only 100 nm in diameter (Ickenstein et al. 2003). The faceted nanostructures are as evident as similar grain structures in monolayers identified by fluorescent lipids that phase separate to the grain boundaries, as studied by Dennis Kim (Kim 1999; Kim and Needham 2001; Kim et al. 2003). Importantly then, the microparticle diameter is ≈5 μm with 0.5 μm grains; in the lower panel, the liposome is 100 nm, with 20 nm grains. Thus, grain structure is commensurate with total domain material size.
TABLE 23.3Yield Shear and Shear Viscosity of Solid Membranes
Now comes the experiment that generated the concept for lysolipid modification of the phase transition, to generate such rapid drug release from the thermosensitive liposomes. At the time, we were not studying heat-triggered drug release, but we were studying molecular exchange with lysolipids (Needham et al. 1997). As shown in Table 23.4, by using three micropipettes in the microscope chamber, our very talented postdoc, Natalia Stoicheva, could hold a single-test vesicle with one pipette and then introduce one of the two flow pipettes in order to either deliver a lysolipid solution (the lysolipid was MOPC) or, after such delivery, wash the vesicle with MOPC-free solution at controlled flow rates. As shown in Table 23.4a, the initial vesicle projection length (Lp) inside the pipette is established when the lower pipette is used to flow MOPC-free bathing solution over the vesicle. Then, the lower pipette is replaced by the upper pipette, and MOPC solution is made to flow over the vesicle, causing an increase of the vesicle projection length, ∆Lp (Table 23.4b). This ∆Lp then gives the membrane area change due to individual molecules of lysolipid entering the outer monolayer of the vesicle, and the plot shows uptake of MOPC monomer reaching 6 mol% before the MOPC-free bathing solution rapidly washes back out the MOPC.
TABLE 23.4Molecular Exchange with Lysolipids
Source: Reproduced from Biophys. J., 73(5), Needham, D., Stoicheva, N., and Zhelev, D.V., Exchange of monooleoylphosphatidylcholine as monomer and micelle with membranes containing poly(ethylene glycol) lipid, 2615–2629. Copyright 1997, with permission from Elsevier.
So, what was the idea? “If lysolipid can be included in a liquid bilayer and then trapped in the bilayer in its gel state, would it desorb or form defects that would enhance the release of encapsulated membrane-impermeable contents?” The answer is yes, as described in Section 23.3.2.
Finally, the whole story about stabilizing vesicle–vesicle adhesive contact and aggregation, as well as protein binding due to a stealth effect (as opposed to mechanical stiffness), starts with fundamental measurements and theory of what it is to be adherent and what it is to be stabilized against adhesion, i.e., the accumulation of all the adhesive and repulsive potentials involving colloidal long-range forces (Evans and Needham 1987, 1988). This is what we were studying in the mid-1980s: by bringing two lipid vesicles together in salt solutions. Much of this work is described in a series of papers with Evans, where we completely stripped back the nature of the attractive and repulsive potentials at lipid bilayer interfaces and introduced them one at a time: measure one thing (adhesion energy) and change one thing (interactive potentials by changing the lipid, salt solution concentration, or polymer composition) in the system. In 1980, Evans had already modeled the vesicle adhesion experiment and trialed some preliminary systems, but again, it was my job to make an experiment that matched exactly his required boundary conditions of enough excess membrane so that—as shown in the Figures in Table 23.5—the left-hand vesicle tension could be gently lowered, allowing the vesicle membrane to spread on the tensioned, right-hand vesicle of the same material (SOPC).
In the experiment, we first measured a fundamental force of nature, van der Waals attraction, limited at PC membrane surfaces by a very short-range hydration repulsion. We then started adding in all the other potentials, as described in Evans and Needham (1987). By adding in charged lipids, this attraction could eventually be overcome by ≈5 mol% phosphatidylserine, which fitted according to the well-known DLVO theory for colloid stability. For neutral vesicles, adding extraneous nonadsorbing polymers, like PEG or dextrans, generated large attractive stresses and highly measured adhesion energies, which again were well modeled by depletion–flocculation theory (Evans and Needham 1988). These attractive potentials were so large they could overcome even mutual negative repulsions at the interfaces of up to 30 mol% charged lipids. Finally, on this baseline, the inclusion of PEG lipids caused separations of the membranes and overcame all long-range colloidal forces and generated the “stealth” effect, as described in more detail with Dan Lasic (Lasic and Needham, 1995).
Although not part of the LTSL story, we (with Dorris Noppl, a student exchange from Germany) used the same two-vesicle experiment to observe and measure receptor-mediated adhesion (Needham and Kim 2000; Noppl-Simson and Needham 1996), an effect that could have some bearing on ligand targeting.
To summarize then, and as described more fully in another recent review (Bagatolli and Needham 2014), these experiments have characterized a two-molecule-thick membrane:
• It is a soft elastic material with a compressibility somewhere between that of a bulk liquid and a gas.
• It is stiffened considerably by the inclusion of cholesterol to levels equivalent to polyethylene.
• It is permeable to water in relation to its compliance.
• It displays a 25% change in area when taken through its main acyl chain freezing transition.
• As a gel-phase material, it shows the yield shear and shear viscosity of a Bingham plastic.
• It can exchange small amounts of other lipids and surfactants with its surrounding milieu.
When manipulated in pairs, lipid vesicles have also been found to be subject to the same range of attractive and repulsive colloidal interactions as many other colloidal particles, including
• Van der Waals attraction, limited by a very-short-range hydration repulsion and the variable-range power law of electrostatic repulsion
• Steric repulsive barriers due to the presence of bound aqueous polymers like PEG, incorporated as, for example, PEG lipid
• Depletion and flocculation by water-soluble polymers like PEG and dextrans when free in surrounding solution
• Intimate mixing (fusion) when manipulated into contacts that reduce the hydration barrier and allow membrane–membrane fusion
DMPC is not much smaller (2× (CH2)) than DPPC, the lipid used in ThermoDox®. And so, even in 1983–1987 (over 10 years before I invented the LTSL), these thermomechanic studies of GUVs were solidifying in my mind exactly what happens when a liposome is taken through a phase transition by changing the temperature, in terms of its physical behavior, the membrane area change, the broadening by a second lipid or cholesterol component, and the shear and viscosity as a result of sliding grain boundaries in the membrane surface. All that was needed now to provide the “light bulb” of the LTSL idea was a fundamental understanding of what happens when a lipid vesicle is exposed to a soluble lipid that can partition into the membrane and can be washed out again, upon changing the bathing medium.
This section may seem arduous, in the context of a chapter on commercialization, but it is absolutely necessary, in order to create survivable patents and to understand the mechanism that the technology is based on (as opposed, again, to patenting a whole series of compositions and not having much clue at all about CSP relationships that influence performance and indeed are part of processing). This then is the level of understanding that is needed, in order to fully characterize your nanomedicine and develop one that actually works in the way it was designed to. I would, therefore, gently suggest that you might need at least some of the same kinds of insights, if you are going to invent anything of use in nanomedicine. There is another lesson to be learned here (see Box 23.3)
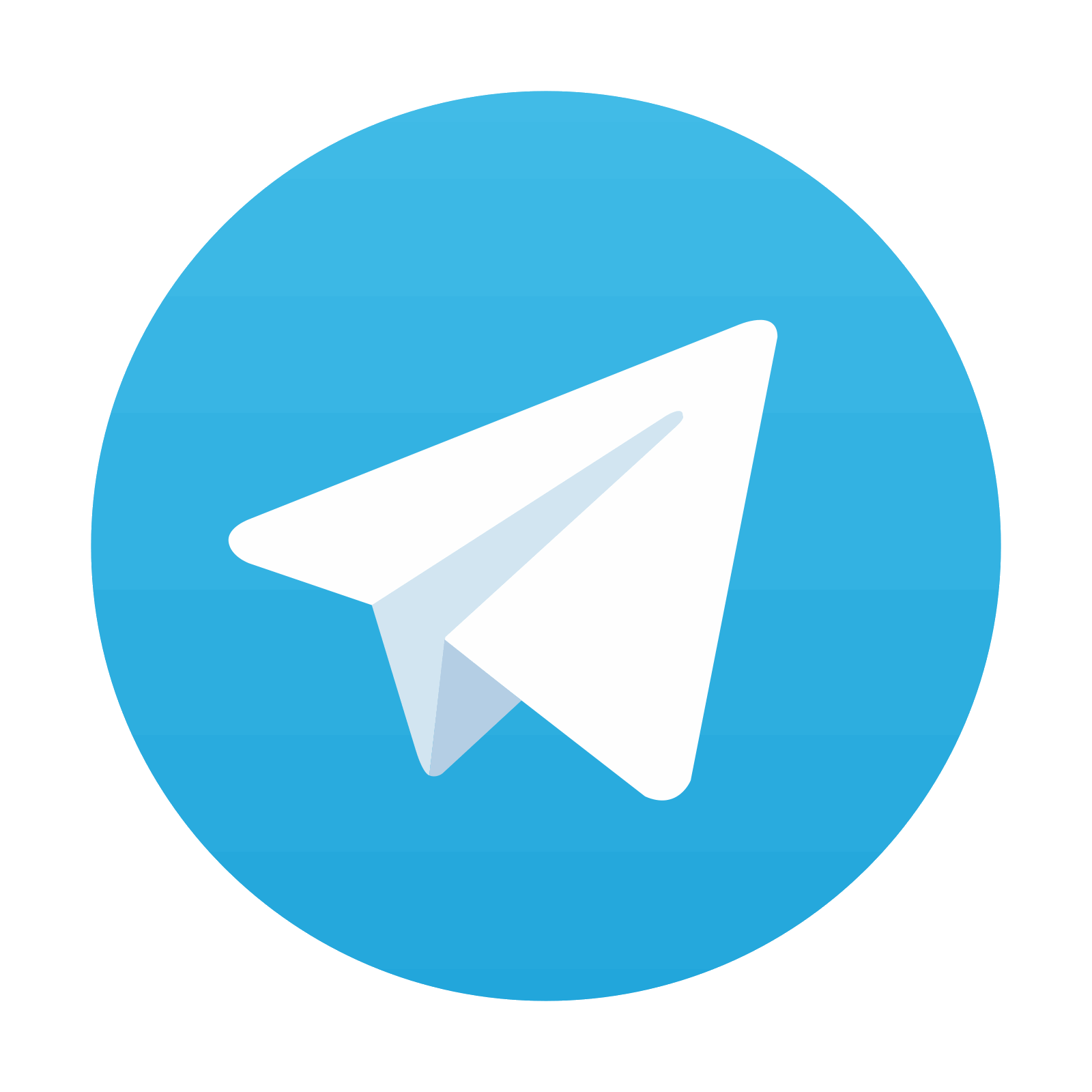
Stay updated, free articles. Join our Telegram channel
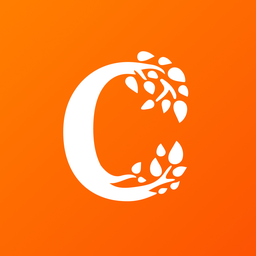
Full access? Get Clinical Tree
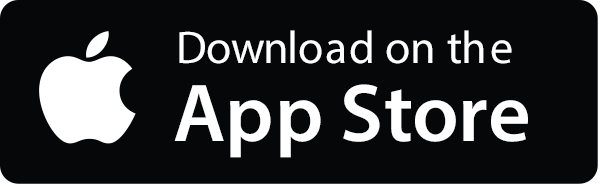
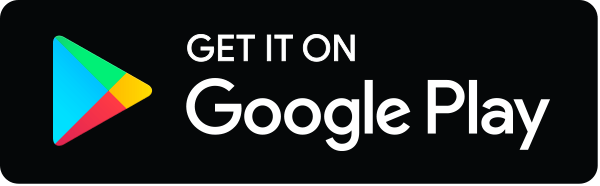