(Eq. 17-1)
Through metabolism, the body produces approximately 150 g of H+ each day. Yet the concentration of H+ in the extracellular body fluids is maintained within a narrow range from 36 to 44 nmol/L (pH 7.35 to 7.45). Through mechanisms that involve the lungs and kidneys, the body controls and excretes H+ in order to maintain pH homeostasis. Imbalances between the rate of acid formation and excretion can occur and can lead to alterations in consciousness, neuromuscular irritability, tetany, coma, and death.
The relative strengths of acids and bases, their ability to dissociate in water, are described by their dissociation constant (Ka). Tables of dissociation constants can be found online and in most biochemistry texts. The pKa is defined as the negative log of the dissociation constant. The dissociation constant is also known as the ionization constant. If the pH equals pKa, then the solution in equilibrium and protonated and unprotonated species are present in equal concentrations. If the pH of the solution is less than pKa then majority of the components will largely be protonated. Many species have more than one pKa meaning they can accept or donate more than one H+.
Buffer Systems: Regulation of H+ and the Henderson-Hasselbalch Equation
A buffer consists of a weak acid and a salt of its conjugate base, and it resists the change in pH upon adding acid or base. The effectiveness of a buffer depends on the pKa of the buffering system and the pH of the environment in which it is placed. In plasma, the bicarbonate–carbonic acid system, is one of the principal buffers:
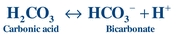
Buffers consist of a weak acid, such as carbonic acid (H2CO3), and a salt of its conjugate base, such as bicarbonate (HCO3–), which forms the bicarbonate–carbonic acid buffer system. H2CO3 is a weak acid because it does not completely dissociate into H+ and HCO3–, whereas a strong acid, such as HCl, completely dissociates into H+ and Cl– in solution. When hydrogen ions are added to the bicarbonate–carbonic acid system, the HCO3– will combine with the H+ to form H2CO3 (Eq. 17-2). Therefore, bicarbonate–carbonic acid reaction is driven to the right, increasing the amount of carbonic acid and consuming bicarbonate ion. Conversely when a strong base is added, H2CO3 will combine with the OH– ions to form H2O and the weaker conjugate base HCO3–.
Although the bicarbonate–carbonic acid system has low buffering capacity, it is still the most important buffer system in extracellular fluids for three reasons: (1) H2CO3 dissociates into CO2 and H2O, allowing CO2 to be eliminated by the lungs and H+ as water; (2) changes in CO2 modify the ventilation (respiratory) rate; and (3) HCO3– concentration can be changed by the kidneys.
Proteins and phosphates are also involved in buffering, primarily in the intracellular fluids and to a minor extent in the extracellular spaces. Most circulating proteins have a net negative charge and are capable of binding H+. The phosphate buffer system (HPO42– – H2PO4–) is the primary buffer in urine and is involved in the exchange of sodium ion in the urine filtrate. Hemoglobin plays a role in buffering the CO2 as it is transported to the lungs for excretion.
The lungs and kidneys play important roles in extracellular fluid pH homeostasis. The interrelationship of the lungs and kidneys in maintaining pH is depicted by the Henderson-Hasselbalch equation (Eq. 17-3). The Henderson-Hasselbalch equation expresses acid–base relationships in a mathematical formula:
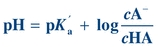
where A– is the proton acceptor or base (e.g., HCO3–), HA is the proton donor or weak acid (e.g., H2CO3), and pKa′ is the pH at which there is an equal concentration of protonated and unprotonated species. Knowing any of the three variables allows for the calculation of the fourth.
Regulation of Acid–Base Balance: Lungs and Kidneys (Transport of Carbon Dioxide)
Carbon dioxide, the end product of most aerobic metabolic processes, easily diffuses out of the tissue where it is produced and into the plasma and red cells in the surrounding capillaries. In plasma, a small amount of CO2 is physically dissolved or combined with amino groups of proteins to form carbamino compounds. However, most of the CO2 freely diffuses into the red blood cells and catalysed by carbonic anhydrase, combines with H2O to form H2CO3, that dissociates into H+ and HCO3– (Fig. 17.1).
FIGURE 17.1 Interrelationship of the bicarbonate and hemoglobin buffering systems.
The dissociation of H2CO3 causes development of a concentration gradient due to the increase in HCO3– concentration; accordingly, biocarbonate ions diffuse from the red cells and diffuse into the plasma. To maintain electroneutrality (the same number of positively and negatively charged ions on each side of the red cell membrane), chloride diffuses into the cell. This is known as the chloride shift (also known as the Hamburger shift). The H+ generated is buffered by binding with the recently deoxygenated hemoglobin molecules. This is why the abbreviation HHb is used to designate deoxygenated hemoglobin, because it has hydrogen bound instead of oxygen.
In the lungs, this process is reversed. Inspired O2 diffuses from the alveoli into the blood and is bound to hemoglobin, forming oxyhemoglobin (O2Hb). The H+ that was carried on the (reduced) hemoglobin in the venous blood is released to recombine with HCO3– to form H2CO3, which dissociates into H2O and CO2. The CO2 diffuses into the alveoli and is eliminated through ventilation. The net effect of these systems is a minimal change in H+ concentration between the venous and arterial circulation. When the lungs do not remove CO2 at the rate of its production, it accumulates in the blood, causing an increase in H+ concentration. However, if CO2 removal is faster than its production (hyperventilation), the H+ concentration will be decreased. Therefore, the pH of the blood will increase. A change in the H+ concentration of blood that results from nonrespiratory disturbances causes the respiratory center to respond by altering the rate of ventilation in an effort to restore the blood pH to normal. The lungs together with the buffer systems provide the first line of defense to change the acid–base status.
The kidneys regulate the excretion of both acid and base, making them an important player in the regulation of acid–base balance. Specifically, the kidney’s main role is to reabsorb bicarbonate ion, HCO3–, from the glomerular filtrate in the proximal tubules (Fig. 17.2A). If bicarbonate ions were not reasborbed and excreted in the urine, this would impact the bicarbonate–carbonic acid buffer system resulting causing a markedly increased levels of H+ ions. Because the glomerular filtrate contains the same HCO3– concentration as plasma, passive reabsorption of HCO3– across the tubule membrane into the blood cannot occur. Instead, sodium (Na+) in the glomerular filtrate is exchanged for H+ in the tubular cell. The H+ combines with HCO3– in the filtrate to form H2CO3, that is subsequently converted into H2O and CO2 by carbonic anhydrase. The CO2 diffuses into the tubular cells and reacts with H2O to reform H2CO3, which then largely disassociates into hydrogen and bicarbonate ions. The bicarbonate ion is reabsorbed into the blood along with sodium. The hydrogen ion provides a vehicle to reabsorb bicarbonate ion. In a healthy individual, virtually all of the filtered bicarbonate ion is reabsorbed.
FIGURE 17.2 Bicarbonate reabsorption by the proximal tubule cell. C–A, carbonic anhydrase.
During alkalosis, the kidney excretes HCO3– to compensate for the elevated blood pH. The exchange between H+ and Na+ suggests, in part, why clinicians order pH and blood gases together, along with electrolytes (Na+, K+, and Cl–) to assess the acid–base status of a patient.
Under normal conditions, the body produces a net excess (50 to 100 mmol/L) of acid (H+) each day that must be excreted by the kidney. The minimum urine pH that can be generated is 4.6, which limits the amount of free hydrogen ion excretion. The phosphate buffering system (Fig. 17.2B) allows for greater hydrogen ion excretion. Most of the H+ ions in the glomerular filtrate combine with monohydrogen phosphate (HPO42–) and ammonia (NH3) and is excreted in the urine as dihydrogen phosphate (H2PO4–) and ammonium ions (NH4+). Ammonia is produced by the deamination of glutamine and other amino acids. During acidosis ammonia production is increased to help buffer the excess hydrogen ions.
ASSESSMENT OF ACID–BASE HOMEOSTASIS
The Bicarbonate Buffering System
In assessing acid–base homeostasis, components of the bicarbonate buffering system are measured and calculated. Inferences can be made from these results about the systems produce, retain, and excrete the acids and bases. For the bicarbonate–carbonic acid buffer system, the dissolved CO2 (dCO2) is in equilibrium with CO2 gas, which can be expelled by the lungs. Because the CO2 can be removed, the bicarbonate buffering system is referred to as an open system, and the dCO2, expelled by the lungs, is the respiratory component. The lungs participate rapidly in the regulation of blood pH through hypoventilation or hyperventilation. The kidneys, the nonrespiratory or also known as the metabolic component, control the bicarbonate concentration.
In plasma and at body temperature (37°C), the pKa′ of the bicarbonate–carbonic acid buffer system is 6.1. The concentration of H2CO3 is proportional to the partial pressure exerted by the dCO2. In plasma at 37°C, the value for the combination of the solubility constant for pCO2 and the factor to convert mm Hg to mmol/L is 0.0307 mmol/L/mm Hg. The solubility constant is dependent on temperature and the solvent. Both pH and pCO2 are measured in blood gas analysis, and the pKa′ is a constant; therefore, HCO3– can be calculated:
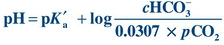
When the kidneys and lungs are functioning properly, the ratio of HCO3– to H2CO3 is 20:1, corresponding to a pH of 7.40. This can be calculated by substituting normal values (Table 17.1) for HCO3– and pCO2 from the preceding equation:
TABLE 17.1 Arterial Blood Gas Reference Range at 37°C
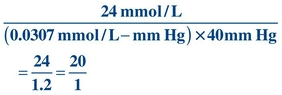
The normal pH of 7.4 can be derived by adding the log of 20 (1.3) to the pKa′ of the bicarbonate system (7.40 = 6.1 + 1.3).
The numerator (HCO3–) denotes the kidney function, whereas the denominator (pCO2, which represents H2CO3) denotes the lung function. The lungs will impact pH through retention or elimination of CO2 by changing the rate and volume of ventilation. The kidneys regulate pH by excreting acid, primarily in the ammonium ion, and by reclaiming HCO3– from the glomerular filtrate.
CASE STUDY 17.1
A 50-year-old man came to the emergency department after returning from foreign travel. His symptoms included persistent diarrhea over the past 3 days and rapid respirations (tachypnea). Blood sample for blood gas analysis was drawn with the following results:
Question
1. What is the patient’s acid–base status?
2. Why is the HCO3– level low?
3. Why does the patient have rapid respirations?
Acid–Base Disorders: Acidosis and Alkalosis
Acid–base disorders result from imbalances in the acid base equilibrium. When blood pH is less than the reference range (7.35 to 7.45), it is termed acidemia. Similarly, a pH greater than the reference range is termed alkalemia. Acidemia will result if the hydrogen ion concentration increases through increased pCO2 concentrations or decreases in the bicarbonate concentration. Alkalemia will result with hydrogen ion concentrations that are decreased, either from decreased pCO2 or increased concentrations of bicarbonate. Technically, the suffix -osis refers to a process in the body; the suffix -emia refers to the corresponding state in blood (-osis is the cause of the -emia).
A disorder caused by ventilatory dysfunction (a change in the pCO2, the respiratory component) is termed primary respiratory acidosis or alkalosis. A disorder resulting from a change in the bicarbonate level (a renal or metabolic function) is termed a nonrespiratory or metabolic disorder.
The response to maintain acid–base homeostasis is termed compensation and is accomplished by altering the factor not associated with the primary process. Mixed acid–base disorders arise from the presence of more than one process or compensatory mechanism in response to the primary disorder. For example, if the imbalance is of metabolic (nonrespiratory) origin, the body compensates by altering ventilation. Conversely, for disturbances of the respiratory component, the kidneys will compensate by selectively excreting or reabsorbing specific ions.
The lungs can immediately compensate by retaining or expelling carbon dioxide; however, this response is short term and often incomplete, whereas the kidneys are slower to respond (2 to 4 days), but the response is long term and sustained. Fully compensated status implies that the pH has returned to the normal range (the ratio of HCO3– to H2CO3 of 20:1 has been restored); partially compensated implies that the pH is approaching normal.
Acidosis may be caused by a primary metabolic or respiratory imbalance. In primary metabolic acidosis, the amount of acid exceeds the capacity of the buffer systems, and there is a decrease in bicarbonate to less than 24 mmol/L. The resulting decrease in pH is due to the decreased ratio of the metabolic to respiratory component in the Henderson-Hasselbalch equation:
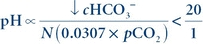
where N is a normal value and ∝ indicates proportional.
The body compensates for metabolic acidosis through hyperventilation, which is an increase in the rate or depth of breathing. By expelling (i.e., “blowing off”) CO2, the base-to-acid ratio will normalize by reducing the pCO2 and thereby elevating the pH. Secondary compensation occurs when the kidneys begin to correct the ratio by excreting hydrogen ions and reabsorbing bicarbonate ions.
Metabolic acidosis may be caused by the following reasons:
- Direct administration or ingestion of acid-producing substances (i.e., ammonium chloride, calcium chloride, salicylates, ethanol).
- Production of organic acids as seen with diabetic ketoacidosis (increased levels of acetoacetic acid and β-hydroxybutyric acid and lactic acid in lactic acidosis).
- Reduced excretion of acids as seen in renal tubular acidosis.
- Excessive loss of bicarbonate from diarrhea or drainage from a biliary, pancreatic, or intestinal fistula.
CASE STUDY 17.2
An 80-year-old woman fell on the ice and fractured her femur. After several hours, upon arrival to the emergency department, she was anxious, panting, and complaining of severe chest pain along with not being able to breathe. Her pulse was rapid (tachycardia) as was her respiration rate (tachypnea). A sample for blood gases was drawn and yielded the following results:
Question
1. What is the patient’s acid–base status?
2. Why is the HCO3– level low?
Primary respiratory acidosis results from a decrease in alveolar ventilation (hypoventilation), causing a decreased elimination of CO2 by the lungs:
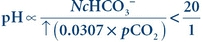
Respiration is regulated in the medulla of the brain. Chemoreceptors present in the aortic arch and the carotid sinus respond to the levels of H+ (pH), O2, and CO2 in the blood and cerebrospinal fluid.
Respiratory acidosis may be caused by the following reasons:
- Ineffective removal of CO2 from the blood as seen in lung disease.
- Airway obstruction as seen in chronic obstructive pulmonary disease (COPD). Destructive changes in the airways and alveolar walls increase the size of the alveolar air spaces, resulting in a reduction of the lung surface area available for gas exchange. As a result, CO2 is retained in the blood, causing chronic hypercarbia (elevated pCO2).
- Drugs such as barbiturates, morphine, and alcohol will cause hypoventilation which subsequently increase blood pCO2 levels.
- Decreased cardiac output, as observed with congestive heart failure, will result in less blood being presented to the lungs for gas exchange and, therefore, an elevated pCO2.
In primary respiratory acidosis, the compensation occurs through metabolic processes. However, as previously mentioned, the metabolic processes, unlike the respiratory, require hours to days to affect the pH. Therefore, it takes days to weeks for full compensation to occur. The kidneys respond to respiratory acidosis by increasing the excretion of H+ and reclamation of HCO3–. As a result of this, HCO3– in the blood increases causing the pH to return to normal.
As with acidosis, alkalosis can result from metabolic and respiratory causes. Primary metabolic alkalosis results from a gain in HCO3–, causing an increase in the pH:
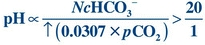
Metabolic alkalosis maybe caused by the following reasons:
- Excess administration of sodium bicarbonate or through ingestion of bicarbonate-producing salts, such as sodium lactate, citrate, and acetate.
- Excessive loss of acid through vomiting, nasogastric suctioning, or prolonged use of diuretics that augment renal excretion of H+ can produce an apparent increase in HCO3–.
- Hypokalemia and chloride deficit stimulate the reabsorption of HCO3– in the distal tubules.
The hydrogen ion sensors in the brain signal the respiratory center to depress respiration, which results in hypoventilation and increases the retention of CO2 thereby increasing pCO2 and lowering the pH. However, this response is erratic, and increases in pCO2 is a stimulus itself to increase respiration. Ultimately, the primary cause of metabolic alkalosis needs to be corrected.
Primary respiratory alkalosis from an increased rate of alveolar ventilation causes excessive elimination of CO2 by the lungs:
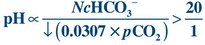
The causes of respiratory alkalosis include:
- Hypoxemia- and hysteria-induced hyperventilation.
- Chemical stimulation of the respiratory center by drugs, such as salicylates and nicotine that cause hyperventilation.
- Pulmonary emboli or pulmonary fibrosis in which pulmonary oxygen exchange is impaired.
The kidneys compensate by excreting HCO3– in the urine and reclaiming H+ to the blood.
CASE STUDY 17.3
A 24-year-old graduate student was brought to the emergency department in a comatose state after being found unconscious in his room. A bottle of secobarbital (sedative) was found on his bed stand. He did not respond to painful stimuli, his respiration was barely perceptible, and his pulse was weak. A sample for blood gases was drawn and yielded the following results:
Question
1. What is the patient’s acid–base status?
2. What caused the profound hypoventilation?
3. Once the respiratory component returns to normal, what will be the patient’s expected acid–base status?
CASE STUDY 17.4
A 24-year-old Himalayan man was accepted to graduate school in the United States. Before leaving home, he had an extensive physical exam that included various blood tests. When the medical staff at the US university reviewed his medical records, it was noted that all test results were normal except the HCO3–, which was 15 mmol/L (reference range, 22 to 26 mmol/L). The HCO3– was done separately on a serum sample. It was not part of a blood gas panel. To rule out nonrespiratory acidosis, the university physician wanted to check current status of the HCO3–. The value was 24 mmol/L.
Question
1. How would his blood gases results (pH, pCO2, HCO3−) differ between home and the US?
2. Which direction does the oxygen dissociation curve shift at altitude?
OXYGEN AND GAS EXCHANGE
Oxygen and Carbon Dioxide
The role of oxygen in metabolism is crucial to life. In the mitochondria, electrons from the oxidation of NADH and FADH2 are transferred through the electron transport chain to reduce molecular oxygen to water. pO2 is measured to evaluate a patient’s oxygen status and is typically measured along with pH and pCO2 in routine blood gas analysis.
For adequate tissue oxygenation, the following seven conditions are necessary: (1) available atmospheric oxygen, (2) adequate ventilation, (3) gas exchange between the lungs and arterial blood, (4) binding of O2 onto hemoglobin, (5) adequate hemoglobin, (6) adequate blood flow to tissues, and (7) release of O2 to the tissue. Any disturbances in these conditions can result in hypoxia, poor tissue oxygenation.
The amount of O2 available in atmospheric air depends on the barometric pressure (BP) and altitude. At sea level, the BP is 760 mm Hg. (In the International System of Units, 1 mm Hg = 0.133 kPa, where 1 Pa = 1 N/m2.) Dalton’s law states that total atmospheric pressure is the sum of the partial pressures of each of the gases in the atmosphere. One atmosphere exerts 760 mm Hg of pressure and is made up of O2 (20.93%), CO2 (0.03%), nitrogen (78.1%), and inert gases (~1%). The percentage for each gas is the same at all altitudes; the partial pressure for each gas in the atmosphere is equal to the BP at a particular altitude times the appropriate percentage for each gas. The vapor pressure of water (47 mm Hg) at 37°C must be accounted for in calculating the partial pressure for the individual gases (Fig. 17.3). In the body, these gases are always fully saturated with water. For example:
FIGURE 17.3 Gas content in lungs and pulmonary and systemic circulation.
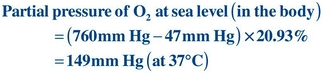
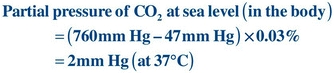
Air is moved into the lungs through expansion of the thoracic cavity, which creates a temporary negative pressure gradient, causing air to move into the numerous tracheal branches that terminate at the alveoli. Gas exchange between alveolar air and pulmonary blood occurs in the alveoli. At the beginning of inspiration, these airways are still filled with air (gas) retained from the previously expired breath. This air, termed dead space air, dilutes the air being inspired. The inspired air is warmed to 37°C and becomes saturated with water vapor and mixes with air in the alveoli. The resulting pO2 in the alveoli averages about 110 mm Hg instead of the anticipated 149 mm Hg. There are many factors that can influence the amount of O2 that moves to the alveoli, and some of the factors are as follows:
- Destruction of the alveoli. The normal surface area of the alveoli is as big as a tennis court. Diminished surface area in diseases such as emphysema will cause an inadequate amount of O2 to move into the blood.
- Pulmonary edema. Gas diffuses from the alveoli into the capillary through a small space. With pulmonary edema, fluid “leaks” into this space and inhibits diffusion of gases.
- Airway blockage. In patients with asthma or bronchitis, airways are blocked, which prevents air from the atmosphere from reaching the alveoli.
There are factors that can influence the amount of O2 to the tissue, and some common causes of inadequate perfusion are as follows:
- Inadequate blood supply. When the blood supply to the lung is inadequate, O2 enters the blood in the lungs, but not enough blood is being carried away to the tissue where it is needed. This may be the consequence of a blockage in a pulmonary blood vessel (pulmonary embolism), pulmonary hypertension, or heart failure.
- Diffusion of CO2 and O2. O2 diffuses 20 times slower than CO2, it is more sensitive to problems with diffusion. In instances such as pulmonary edema, hypoxemia will develop but with minimal alteration to pCO2. This type of hypoxemia is generally treated with supplemental O2. The percentage of O2 can be increased temporarily when needed; however, 60% or higher O2 concentrations must be used with caution because it can be toxic to the lungs.
- Intrapulmonary shunting. Nonfunctional alveoli prohibits venous blood from being oxygenated that results in decreased oxygen content.
- Anemia. Blood with less hemoblogin will carry proportionally less oxygen.
Oxygen Transport
Most O2 in arterial blood is transported to the tissue by hemoglobin. In the adult, hemoglobin (A1) molecule can combine reversibly with four molecules of O2. The actual amount of O2 loaded onto hemoglobin depends on the availability of O2; concentration and type(s) of hemoglobin present; presence of competing molecules, such as carbon monoxide (CO); pH; temperature of the blood; and levels of pCO2 and 2,3-diphosphoglycerate (2,3-DPG). With adequate atmospheric and alveolar O2 available and normal diffusion of O2 to the arterial blood, more than 95% of “functional” hemoglobin (hemoglobin capable of reversibly binding O2) will bind O2. The percentage of O2 in inspired air, or fraction of inspired oxygen (FiO2), can be increased by breathing gas mixtures up to 100% O2. Increasing FiO2 further saturates hemoglobin. However, once the hemoglobin is 100% saturated, an increase in O2 to the alveoli serves only to increase the concentration of dO2 in the arterial blood. Prolonged administration of high concentrations of O2 may cause oxygen toxicity and, in some cases, decreased ventilation that leads to hypercarbia.
Hemoglobin exists in one of four conditions:
1. O2Hb is the erythrocyte hemoglobin. Ferrous iron (Fe2+) in the heme group reversibly binds to O2.
2. Deoxyhemoglobin (HHb), also known as reduced hemoglobin, is hemoglobin not bound to O2 but capable of forming a bond when O2 is available. Notably, hemoglobin increases its affinity to hydrogen ions as it loses O2.
3. Carboxyhemoglobin (COHb) is hemoglobin bound to carbon monoxide (CO) and is not available for O2 transport. This is because the bond between CO and Hb is 200 times stronger than the bond between O2 and Hb.
4. Methemoglobin (MetHb) is hemoglobin unable to bind O2 because iron (Fe) is in an oxidized (Fe3+) rather than reduced state. The Fe3+ can be reduced by the enzyme methemoglobin reductase, which is found in red blood cells.
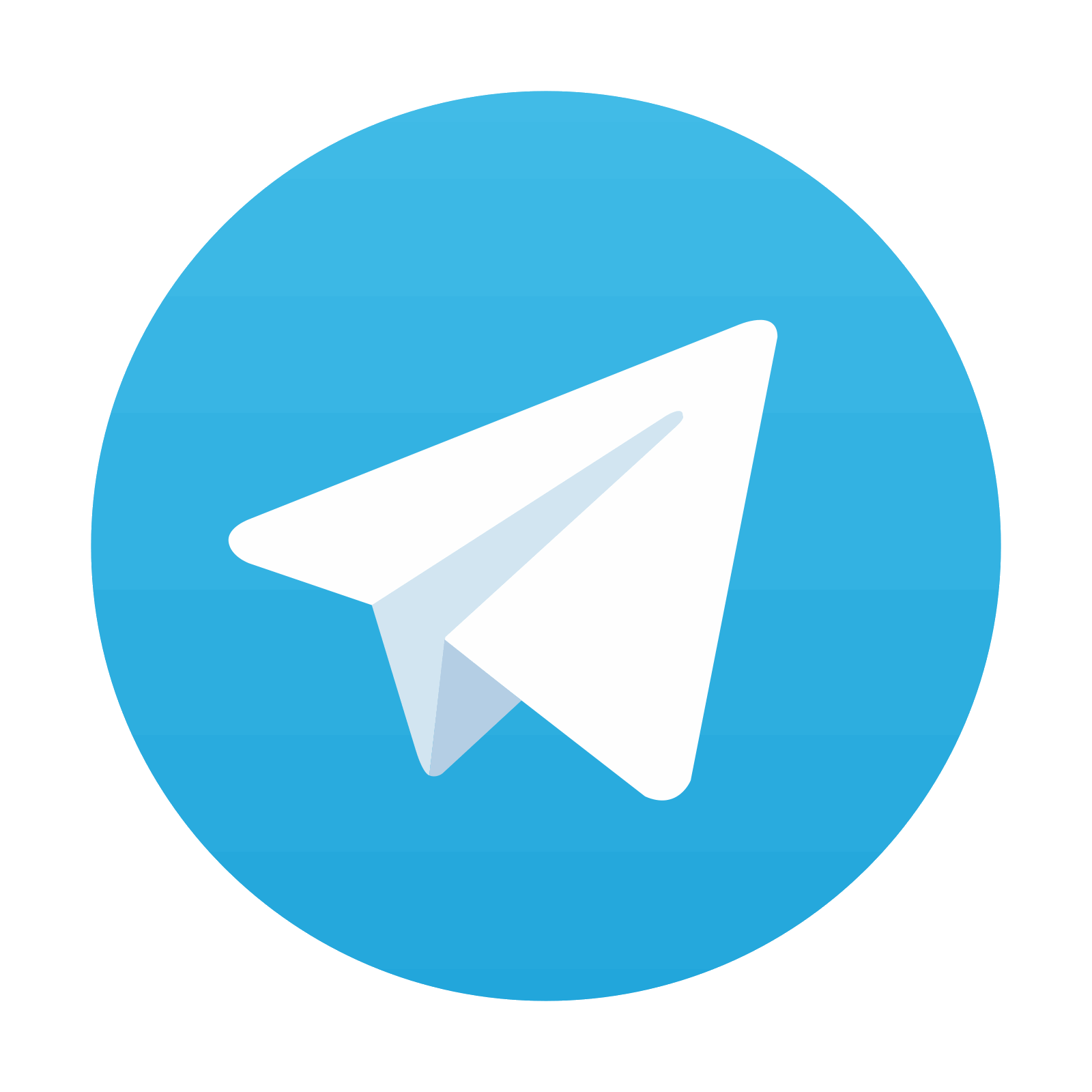
Stay updated, free articles. Join our Telegram channel
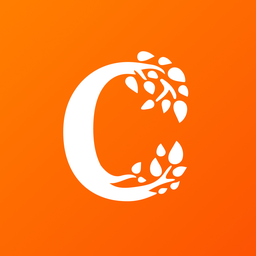
Full access? Get Clinical Tree
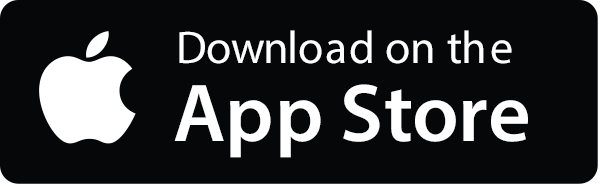
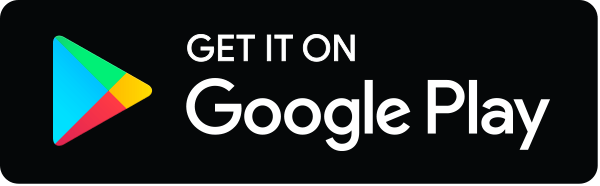