Biotin1
Donald M. Mock
1Abbreviations: ACC, acetyl-CoA carboxylase; AMP, adenosine monophosphate; CoA, coenzyme A; HCS, holocarboxylase synthetase; MCC, methylcrotonyl-CoA carboxylase; Na+, sodium; PC, pyruvate carboxylase; PCC, propionyl-CoA carboxylase; SMVT, sodium-dependent multivitamin transporter.
HISTORY OF DISCOVERY
Although a growth requirement for the “bios” fraction had been demonstrated in yeast, Boas first demonstrated the mammalian requirement for a factor—biotin—in rats fed egg-white protein. The severe dermatitis, hair loss, and neuromuscular dysfunction were termed “egg-white injury” and were cured by a factor present in liver. The critical event in this egg-white injury of both humans and rats is the highly specific and very tight binding (dissociation constant = 10-15M) of biotin by avidin, a glycoprotein found in egg white. From an evolutionary standpoint, avidin probably serves as a bacteriostat in egg white; consistent with this hypothesis is the observation that avidin is resistant to a broad range of bacterial proteases. Because avidin is also resistant to pancreatic proteases, dietary avidin binds dietary biotin and biotin synthesized by intestinal microbes and thus prevents absorption. Cooking denatures avidin and renders it susceptible to digestion and unable to interfere with absorption of biotin.
STRUCTURE, CHEMISTRY, AND BIOCHEMISTRY
Structure
Biotin is a bicyclic compound (Fig. 28.1). One ring contains a ureido group; the other contains sulfur and has a valeric acid side chain. The structure of biotin was elucidated independently by Kogl and by du Vigneaud in the early 1940s (1). Eight stereoisomers exist, but only one (designated d-[+]-biotin or, simply, biotin) is found in nature and is enzymatically active.
Regulation
In mammals, biotin serves as an essential cofactor for five carboxylases, each of which catalyzes a critical step in intermediary metabolism. Biotin exists in free and bound pools within the cell that are responsive to changes in biotin status (2). The pool size is likely determined by a balance among cellular uptake, cellular release, incorporation into apocarboxylases and histones, release from these biotinylated proteins during turnover, and catabolism to inactive metabolites.
Attachment of biotin to the apocarboxylase (see Fig. 28.1) is a condensation reaction catalyzed by holocarboxylase synthetase (HCS). An amide bond is formed between the carboxyl group of the valeric acid side chain of biotin and the ε-amino group of a specific lysyl residue in the apocarboxylase; these apocarboxylase regions contain sequences of amino acids that tend to be highly
conserved within and among species for the individual carboxylases.
conserved within and among species for the individual carboxylases.
Regulation of intracellular mammalian carboxylase activity by biotin remains to be elucidated. However, the interaction of biotin synthesis and production of holoacetyl-coenzyme A (CoA) carboxylase in Escherichia coli has been extensively studied. In the bacterial system, the availability of the apocarboxylase protein and of biotin (as the intermediate biotinyladenosine monophosphate [AMP]) act together to control the rate of biotin synthesis by direct interaction with promoter regions of the biotin operon, which, in turn, controls a cluster of genes that encode enzymes that catalyze the synthesis of biotin.
In the normal turnover of cellular proteins, holocarboxylases are degraded to biocytin (ε-N-biotinyl-L-lysine) or biotin linked to an oligopeptide containing at most a few amino acid residues (see Fig. 28.1). Biotinidase (biotin amide hydrolase, EC 3.5.1.12) releases biotin for recycling. The clinical manifestations of biotinidase deficiency appear to result largely from a secondary biotin deficiency. The genes for HCS and human biotinidase have been cloned, sequenced, and characterized (3).
Chemistry
All five of the mammalian carboxylases catalyze the incorporation of bicarbonate as a carboxyl group into a substrate and employ a similar catalytic mechanism. In the carboxylase reaction, the carboxyl moiety is first attached to biotin at the ureido nitrogen opposite the side chain; then the carboxyl group is transferred to the substrate. The reaction is driven by the hydrolysis of adenosine triphosphate. Subsequent reactions in the pathways release carbon dioxide. Thus, these reaction sequences rearrange the substrates into more useful intermediates, but they do not violate the classic observation that mammalian metabolism does not result in the net fixation of carbon dioxide (4).
Biotin-Dependent Carboxylases
The five biotin-dependent mammalian carboxylases are acetyl-CoA carboxylase (ACC; EC 6.4.1.2) isoforms I and II, pyruvate carboxylase (PC: EC 6.4.1.1), methylcrotonyl-CoA carboxylase (MCC; EC 6.4.1.4), and propionyl-CoA carboxylase (PCC; EC 6.4.1.3).
Both acetyl-CoA carboxylases catalyze the incorporation of bicarbonate into acetyl-CoA to form malonyl-CoA
(Fig. 28.2). Isoform 1 of ACC (ACC-1) is encoded by the ACACA gene and is located in the cytosol. The malonyl-CoA produced by ACC-1 is rate limiting in fatty acid synthesis (elongation). Isoform 2 of ACC (ACC-2) is encoded by the ACACB gene and is located on the outer mitochondrial membrane. ACC-2 controls fatty acid oxidation in mitochondria through the inhibition of carnitine palmitoyltransferase I by its product malonyl-CoA. Carnitine palmitoyltransferase I catalyzes the rate limiting step in fatty acid uptake into mitochondria and thus regulates the availability of fatty acids for oxidation. Thus, ACC-1 and ACC-2 are thought to have two very different roles in cellular metabolism; one controls fatty acid synthesis, and the other controls fatty acid oxidation. An inactive mitochondrial form of ACC may also serve as storage for biotin (5).
(Fig. 28.2). Isoform 1 of ACC (ACC-1) is encoded by the ACACA gene and is located in the cytosol. The malonyl-CoA produced by ACC-1 is rate limiting in fatty acid synthesis (elongation). Isoform 2 of ACC (ACC-2) is encoded by the ACACB gene and is located on the outer mitochondrial membrane. ACC-2 controls fatty acid oxidation in mitochondria through the inhibition of carnitine palmitoyltransferase I by its product malonyl-CoA. Carnitine palmitoyltransferase I catalyzes the rate limiting step in fatty acid uptake into mitochondria and thus regulates the availability of fatty acids for oxidation. Thus, ACC-1 and ACC-2 are thought to have two very different roles in cellular metabolism; one controls fatty acid synthesis, and the other controls fatty acid oxidation. An inactive mitochondrial form of ACC may also serve as storage for biotin (5).
![]() Fig. 28.2. Interrelationship of pathways catalyzed by biotin-dependent enzymes (boxes). CoA, coenzyme A. |
The three remaining carboxylases are mitochondrial. PC catalyzes the incorporation of bicarbonate into pyruvate to form oxaloacetate, an intermediate in the Krebs tricarboxylic acid cycle (see Fig. 28.2). In gluconeogenic tissues (i.e., liver and kidney), the oxaloacetate can be converted to glucose. Deficiency of PC is probably the cause of lactic acidemia, central nervous system lactic acidosis, and abnormalities in glucose regulation observed in biotin deficiency and biotinidase deficiency (see later).
MCC catalyzes an essential step in the degradation of the branched-chain amino acid leucine (see Fig. 28.2). Deficient activity of MCC leads to metabolism of 3-methylcrotonyl-CoA to 3-hydroxyisovaleryl-CoA, 3-hydroxyisovaleryl-carnitine, and 3-hydroxyisovaleric acid by an alternate pathway (1). Increased urinary excretions of 3-hydroxyisovaleryl-carnitine and 3-hydroxyisovaleric acid reflect deficient activity of MCC and are biomarkers of biotin deficiency (1, 6).
PCC catalyzes the incorporation of bicarbonate into propionyl-CoA to form methylmalonyl-CoA; methylmalonyl-CoA undergoes isomerization to succinyl-CoA and enters the tricarboxylic acid cycle (see Fig. 28.2). In a fashion analogous to MCC deficiency, deficiency of PCC leads to increased urinary excretion of 3-hydroxypropionic acid and 3-methylcitric acid.
Metabolism
In humans, about half of biotin is catabolized to inactive metabolites before excretion in urine (4). The two principal metabolites are bisnorbiotin and biotin sulfoxide. Bisnorbiotin is produced by β-oxidation of the valeric acid side chain. Biotin sulfoxide is produced from oxidation of the sulfur in the thiophane ring. Other minor metabolites result from continued β-oxidation of the side chain, further oxidation of the sulfur, or a combination.
On a molar basis, biotin accounts for approximately half of the total avidin-binding substances in human serum and urine (Table 28.1). During pregnancy and long-term anticonvulsant treatment, accelerated biotin catabolism is thought to contribute to biotin deficiency.
Measurement of Biotin and Metabolites
For measuring biotin at physiologic concentrations (i.e., 100 pmol/L to 100 nmol/L), various assays have been proposed, and a few have been used to study biotin nutriture. For a more detailed review, please refer to the study by Mock (7). Most published studies of biotin nutriture have used one of two basic types of biotin assays: bioassays or avidin-binding assays.
Bioassays generally have adequate sensitivity to measure biotin in blood and urine, especially with modifications using injected agar plates or metabolic radiometry. However, the bacterial bioassays (and perhaps the eukaryotic bioassays
as well) suffer interference from unrelated substances and variable growth response to biotin analogs. Bioassays give conflicting results if biotin is bound to protein (7).
as well) suffer interference from unrelated substances and variable growth response to biotin analogs. Bioassays give conflicting results if biotin is bound to protein (7).
TABLE 28.1 NORMAL RANGE FOR BIOTIN AND METABOLITES IN HUMAN SERUM AND URINEa | |||||||||||||||
---|---|---|---|---|---|---|---|---|---|---|---|---|---|---|---|
|
Avidin-binding assays generally measure the ability of biotin to compete with radiolabeled biotin for binding to avidin (isotope dilution assays), to bind to avidin coupled to a reporter and thus prevent the avidin from binding to a biotin linked to solid phase, or to prevent inhibition of a biotinylated enzyme by avidin. Various novel reporter systems have been described (1, 8). Avidin-binding assays generally detect all avidin-binding substances, although the relative detectabilities of biotin and analogs vary among analogs and among assays (8). High-performance liquid chromatography separation of biotin analogs with subsequent avidin-binding assay of the chromatographic fractions appears to be both sensitive and chemically specific. By using avidin-binding assays, the fasting concentration of biotin in human plasma is approximately 250 pmol/L.
ABSORPTION
Digestion of Protein-Bound Biotin
The content of free biotin and protein-bound biotin in foods is variable, but most biotin in meats and cereals appears to be protein-bound by an amide bond between biotin and lysine.
Intestinal Absorption, Renal Reabsorption, and Uptake by Somatic Cells
At physiologic pH, biotin is at least modestly water soluble and requires a transporter to cross membranes of such cells as enterocytes, somatic cells, and renal tubule cells.
Intestinal Uptake
An excellent in-depth review of intestinal uptake of biotin has been published (9). Human intestinal epithelial cells are highly specialized. Biotin transport must occur across two structurally and functionally different membrane domains: the brush-border membrane that faces the intestinal lumen and the basolateral membrane that faces the interstitium that is in contact with the blood that perfuses the intestine (9).
A biotin transporter is present in each of the membrane domains. In the brush-border membrane, transport occurs through a sodium (Na+)-dependent, electroneutral, carrier-mediated mechanism that saturates at the micromolar range and accounts for the overall limitation in nondiffusion transport (9). In the presence of a Na+ gradient, biotin transport occurs against a concentration gradient. This biotin transporter can also transport pantothenic acid and lipoic acid and hence has been named sodium-dependent multivitamin transporter (SMVT). Human SMVT is the product of the SLC5A6 gene, which is located on chromosome 2p23. SMVT is exclusively targeted to the apical (brush-border) membrane.
Biotin transport across the basolateral membrane is also a carrier-mediated mechanism. However, this carrier is Na+ independent and electrogenic, and it cannot accumulate biotin against a concentration gradient (9).
The intestinal biotin transport up-regulates in response to biotin deficiency in both humans and animal models. The mechanism likely primarily involves induction of SMVT mRNA synthesis and increased number of SMVT transporters per cell. The increase in SMVT is probably mediated by an induction in the activity of P1, which is one of two promoter regions upstream from the SMVT gene (9).
In rats, biotin transport is up-regulated with maturation and by biotin deficiency (10). Although carriermediated transport of biotin is most active in the proximal small bowel of the rat, the absorption of biotin from the proximal colon is still significant—a finding supporting the potential nutritional significance of biotin synthesized and released by enteric flora. However, the quantitative importance of the contribution of enteric biotin synthesis to absorbed biotin remains to be determined.
Based on a study in which biotin was administered orally in pharmacologic amounts, bioavailability of biotin is approximately 100%. Thus, the pharmacologic doses of biotin given to treat biotin-dependent inborn errors of metabolism are likely to be well absorbed. Moreover, the finding of high bioavailability of biotin at pharmacologic doses provides at least some basis for predicting that bioavailability will also be high at the physiologic doses at which the biotin transporter mediates uptake.
Transport from the Intestine to Peripheral Tissues
Biotin is transported in blood from the site of absorption in the intestine to the peripheral tissues and the liver (1). Biotin concentrations in plasma are small relative to other water-soluble vitamins. Most biotin in plasma is free and dissolved in the aqueous phase of plasma. However, approximately 7% is reversibly bound to plasma protein, and approximately 12% is covalently bound to plasma protein. Binding to human serum albumin likely accounts for the reversible binding. Biotinidase has been proposed as a biotin-binding protein or biotin-carrier protein for the transport into cells. Biotin concentrations in erythrocytes are equal to the concentrations in plasma (unpublished observation, D.M. Mock), but transport of biotin into erythrocytes is very slow, consistent with passive diffusion (11).
Uptake by the Liver
SMVT is widely expressed in human tissues. Studies by Subramanya and Said et al, using RNAi specific for SMVT, provided strong evidence that biotin uptake by liver (and likely many other somatic tissues) occurs through SMVT (12). Metabolic trapping, (e.g., biotin bound covalently to intracellular proteins) is also important. After entering the hepatocyte, biotin diffuses into the mitochondria by a pH-dependent process, suggesting that
biotin enters the mitochondria in the neutral, protonated form and dissociates into the anionic form in the alkaline mitochondrial environment, thus becoming trapped by the charge. Based on a study in rats, biliary excretion of biotin is quantitatively negligible.
biotin enters the mitochondria in the neutral, protonated form and dissociates into the anionic form in the alkaline mitochondrial environment, thus becoming trapped by the charge. Based on a study in rats, biliary excretion of biotin is quantitatively negligible.
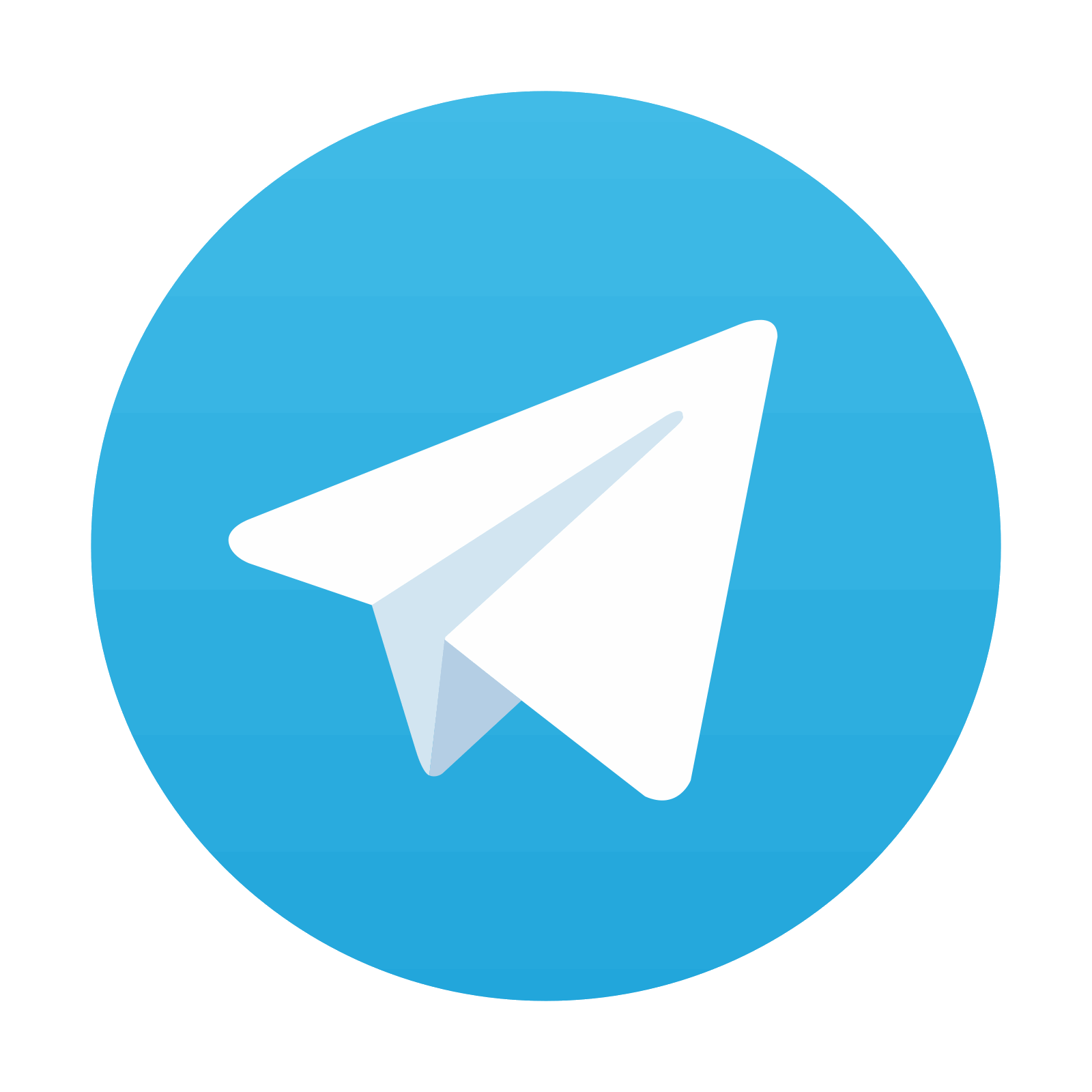
Stay updated, free articles. Join our Telegram channel
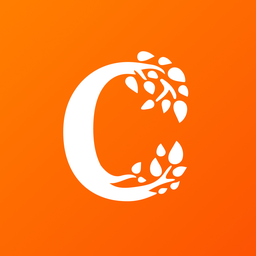
Full access? Get Clinical Tree
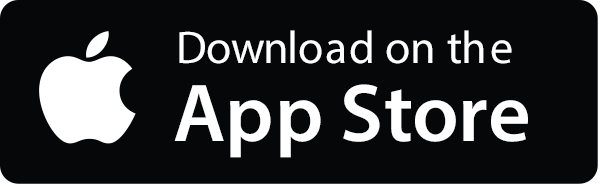
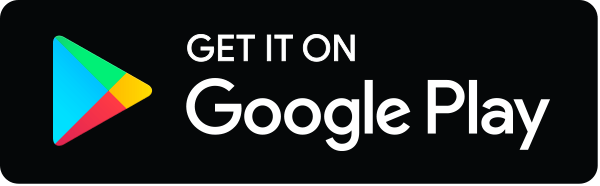