A. SPECIAL REQUIREMENTS FOR BIOMEDICAL MATERIALS
Materials have been used in medical practice since prehistoric times. Today, materials science plays a crucial role in many aspects of biomedical science and technology. Modern biomedical materials fall into two main categories: (1) those selected for their resistance to breakdown in a biological environment and (2) those chosen for their ability to decompose to harmless products when implanted on the body. The field of biostable materials can be further subdivided into solids for hard tissue replacement and those for soft tissue applications. The latter group includes elastomers for cardiovascular applications, hydrogels for optical prostheses, and membranes for controlled drug delivery. Bioerodible (hydrolytically unstable) materials are of interest as tissue regeneration matrices, surgical sutures and screws, and controlled drug delivery devices. These options are summarized in Figure 16.1. Within all these categories are traditional materials that were optimized for some other use, and a few newer substances that have been designed specifically for biomedical applications.
What are the special property requirements for medical materials? The first is the need to avoid any detrimental response by the human body to a “foreign” substance—whether the material is implanted in the body or used in an external device (“extracorporeal”). Satisfying this requirement is not a trivial challenge, as will be seen in later sections of this chapter. The detrimental response may be as basic as rejection of an object because of its shape, roughness, or lack of flexibility, or the response may be based on more complex factors such as toxicity, protein–surface interactions, or colonization by microorganisms. In addition to this primary requirement for biological compatibility, a material must be optimized for a different set of physical properties for each individual medical application. For example, the property combinations needed for a bone reinforcement material are quite different from those required for artificial blood vessels or hemodialysis membranes.
However, the biggest challenge in this field involves the decision between using long-existing materials that are available in large quantities versus newer materials specifically designed to possess a significantly improved set of properties. At present, long-existing materials borrowed from other technologies far outnumber those that have been designed and synthesized primarily for biomedical uses.
The issue of materials stability in the aqueous environment of the human body is an important factor for many applications. Clearly, cardiovascular, optical, and renal devices should ideally last without decomposition for the life of the patient. However, a growing number of medical materials are used because they decompose slowly in water to give harmless products. Absorbable surgical sutures, temporary frameworks for bone, skin, or liver regeneration (“tissue engineering”), and materials for the controlled release of drug molecules or vaccines are examples.
The following section reviews examples of some widely used biomaterials. These are separated into those that are biostable and those that are bioerodible. Later sections deal with the ways in which different materials are used in specific medical applications, followed by some brief observations on fabrication and testing, and comments on some unsolved problems in this field.
Many materials types and their properties that are important in biomedicine are discussed in other chapters. For example, membranes for biomedical applications are described in Chapter 13. Polymers are discussed in Chapter 6. Surfaces that are optimized for biomedical applications are covered in Chapter 15. Orthopedic composites are mentioned in Chapter 9. The purpose of this chapter is to provide an overview of the biomedical materials field, and the reader is encouraged to integrate the material covered elsewhere in this book with the information provided here.
B. TRADITIONAL BIOMEDICAL MATERIALS
Metals, ceramics, and polymers are used as biomedical materials. Of these, polymers are being increasingly employed in medical devices, although metals and ceramics have important specialized applications.
1. Metals
The requirement of corrosion resistance limits the number of metals that can be employed in internal medicine. The commonest examples of metals in use are gold, silver, and silver–mercury alloys (“silver amalgam”), titanium, and stainless steel.
Gold is widely used in tooth restoration because of its inertness, malleability, and ease of fabrication by melting. Modern tooth restorations often use gold bonded to a ceramic outer coating to mimic the appearance of natural tooth enamel. The high cost of gold is, of course, a limiting factor.
Silver is less expensive than gold, but more prone to tarnishing and corrosion. However, when alloyed with mercury, it is one of the most widely used materials for dental fillings. When first mixed, the alloy is moldable at room temperature as it is forced into the tooth cavity, where it sets to a tough antibacterial solid that can last as an effective restoration for 50 or more years. Fears about the long-term release of mercury into the body seem to be unfounded.
Stainless steel has for many years been the material of choice for use as pins and spikes to reinforce bone fractures. It has high strength and is corrosion-resistant. Stainless steel is also heavy, and this is a definite drawback. Some concern exists about the possible long-term release of transition metals such as chromium or nickel into the body, but the beneficial properties are considered to outweigh any potential disadvantage.
Titanium is a metal that overcomes nearly all of these drawbacks. It is lightweight and corrosion-resistant. However, as discussed in Chapter 8, titanium is more difficult to fabricate than stainless steel and is more expensive. Both stainless steel and titanium are employed as the ball joint in artificial hips.
One often-overlooked advantage to the use of metals in medicine is that they are opaque to X rays. Thus, their location and condition can be monitored by conventional X-ray methods. Metals are also easy to sterilize by heat, chemicals, or gamma radiation. A problem with the use of metals in orthopedics is that they tend to trigger warning devices at airports and some public buildings.
2. Ceramics
The main uses for oxide ceramics in medicine are in replacements or fillings for teeth, in bone repair materials, or in artificial hip joints.
Calcium hydroxyapatite (HAP) [Ca10(PO4)6(OH)2] is the mineral component of both bone and tooth enamel (Chapter 7). Indeed, various composites of HAP with polymers have wide use in dental restoration and bone repair cements. HAP-based ceramics can, under special conditions, be bioerodible, but the rate of erosion is very slow. Inorganic composites such as zinc oxide and phosphoric acid are used as tooth fillings. Porcelain tooth restoration and denture ceramics are well known. Glass–ionomer tooth restoration cements are based on silicate–acrylic acid composites.
Nonoxide ceramics such as glassy carbon or silicon carbide have been proposed for both dental and orthopaedic applications, but their use is still limited. Glassy carbon is used in artificial heart valves (see Section C.1).
3. Polymers
The flexibility, elasticity, and ease of fabrication of most polymers make them an obvious choice for many biomedical applications. In physical properties they can be chosen to mimic the characteristics of many different types of living tissues, from soft tissues to bone. In addition, their surfaces can be modified to enhance biocompatibility. Moreover, a few polymers degrade hydrolytically to relatively harmless products.
However, some disadvantages also exist. For example, two of the main lines of attack by the body on foreign objects are hydrolysis and oxidation reactions, and many otherwise biostable polymers are susceptible to oxidative breakdown. Moreover, polymers tend to be more prone to colonization by bacteria or fungi than do metals or ceramics. Another disadvantage of many polymers is that they are too fragile for sterilization by heat. Thus, exposure to γ rays or chemicals is the preferred sterilization method. A major problem for some polymers is that they contain plasticizers and/or monomer or catalyst residues, and leaching of these into the body can give rise to a variety of serious physiological problems. Many of the early evaluations of polymers as biomaterials neglected to take this factor into account.
Nearly all the different classes of polymers have been investigated as bio materials. The descriptions presented in the following paragraphs are intended for purposes of illustration, and are by no means comprehensive. Seemingly biostable polymers are considered first, followed by bioerodible materials. Their structures are shown in Table 16.1.
a. Biostable Polymers
(1) Polyethylene.
This hydrophobic polymer (structure 16.1 in Table 16.1) is used in the socket of artificial hip joints. Its biomedical utility is based on the fact that it is impervious to biological fluids, can be fabricated easily by melt or machining techniques, and appears to be bioinert, at least over the short term. However, surprisingly, given its chemical structure, evidence exists that its molecular weight and strength begin to decrease within months after implantation, possibly by oxidative breakdown.
TABLE 16.1. Examples of Polymers Used in Clinical Practice

(2) Poly(tetrafluoroethylene) (PTFE, Teflon, Gore-Tex).
This polymer (structure 16.2 in Table 16.1) is widely used as an inert biomedical material. It is one of the most hydrophobic polymers known (contact angle to water ~100°), and it is thus resistant to penetration by water or microorganisms. Monolithic Teflon is a relatively inflexible, machinable version of the polymer. Gore-Tex is a flexible, highly porous modification used in arterial replacement tubes. Velours (felt-like) surfaces with PTFE nanofibers are of some interest as platforms for cell spreading. PTFE is one of the few materials that appear to be stable enough in a biological environment to last the lifetime of a patient. The main drawback of PTFE is the difficulty of fabrication since it does not melt below 327°C and it is soluble in various solvents only at temperatures close to its melting point. Thus, sinter fabrication is one of the few alternatives for device preparation.
(3) Poly(methyl methacrylate) (PMMA).
PMMA (structure 16.3 in Table 16.1) was once used in a variety of biomedical devices, but is now employed mainly in hip replacement surgery to cement the metal ball joint spike into the end of the femur, a process that is accomplished by in situ free-radical polymerization of the monomer. Some questions exist about the toxicity of the monomer released into the body during this procedure.
(4) Polyesters.
The principal polyester used in biomedicine is poly(ethylene terephthalate) (structure 16.4 in Table 16.1), also known as Dacron©, Terylene©, or Mylar©. It is a flexible, high-melting-point (258°C) polymer that has good chemical resistance, and is melt-spun into strong fibers or fabricated into clear films. As a woven cloth or a velour, it is a favored material for use in tissue reinforcement.
(5) Polyamides.
Although nylons are produced on a vast scale for textiles, their initial use as biomaterials has been largely discontinued due to their susceptibility to hydrolytic breakdown, often to give toxic products. However, synthetic polypeptides produced by the polymerization of amino acids are useful as bioerodible polymers.
(6) Poly(dimethylsiloxane) (Silicone Rubber) (PDMS).
This polymer (structure 16.6 in Table 16.1) has a long history of successful use as a hydrophobic elastomeric biomaterial. It has been utilized in prosthetic heart valves, catheters, subcutaneous devices for the slow release of steroids, and numerous other applications. PDMS tends to absorb lipids from blood, which causes it to soften over time. The legal issues that surrounded silicone breast implants failed to implicate high-polymeric silicones as toxic species. This polymer is one of the most useful elastomeric biomaterials.
(7) Cellulose Acetate.
This is a long-existing polymer (structure 16.7 in Table 16.1) produced by acetylation of cellulose by acetic anhydride, and it is used as a film-forming material in general technology. Its main use in biomedicine is as a semipermeable membrane in hemodialysis equipment. This is a relatively short-term use, and so issues of long-term hydrolytic and oxidative stability do not arise.
(8) Hydrophobic Polyphosphazenes.
Several of these polymers (structure 16.8 in Table 16.1), especially those with fluoroalkoxy or aryloxy side groups, have generated considerable interest as potential hydrophobic alternatives to silicone rubber or Teflon-type fluorocarbon materials. Fluorinated phosphazene polymers do not absorb lipids, and their structures can be tuned to give either elastomers or film-formers. Unlike Teflon, they are soluble in many organic solvents and can be fabricated easily. Their contact angles to water are higher than that of PDMS and are similar to that of Teflon. As nanofibers, they are even more hydrophobic (~160°). Blood and tissue compatibility tests suggest that they are good candidates for cardiovascular applications, catheters, pacemaker coatings, and related devices. These polymers are examples of newer candidates that show some advantages over traditional materials.
(9) Polyurethanes.
Although some polyurethanes (structure 16.9 in Table 16.1) are unstable to water and oxidation over periods of years, others (especially those with poly(dimethylsiloxane) and alkyl ether block structures) are believed to be biostable. However, their main attribute is elasticity combined with flex strength. Polyurethane films can be flexed millions of times before materials fatigue sets in. Hence, they are used as membranes in blood pumps, and have FDA approval for this application.
(10)Poly(ethylene glycol) (PEG).
This polymer (structure 16.10 in Table 16.1) is a water-soluble, biostable, macromolecule that has limited FDA approval for use in human biomedicine. It is crosslinked to give hydrogels, is used as a viscosity-enhancing agent, and can be attached to surfaces to make them hydrophilic. The safety of this polymer depends on the molecular weight. High polymers cannot be excreted through the kidneys, and they accumulate in the spleen and liver. Low-molecular-weight linear polymers (MW below 1000–2000) are excreted.
(11) Poly(hydroxyethyl methacrylate) (HEMA).
This (structure 16.11 in Table 16.1) is another water-soluble polymer with a long history of use in experimental biomaterials research, especially as a material for conversion to hydrogels. Its hydrogels have been developed for uses in contact lenses and intraocular lenses, and, like PEG, it produces hydrophilic surfaces when grafted onto other materials.
(12) Poly(vinyl pyrolidone) (PVP).
PVP (structure 16.12 in Table 16.1) is a water-soluble, hydrolytically stable macromolecule that was used in Germany as a blood plasma substitute during World War II. Because it in nonbioerodible it accumulates in the spleen and liver, but with seemingly benign results. Its main uses today are in semipermeable membranes and in cosmetic products.
(13) Water-Soluble Polyphosphazenes.
Phosphazene polymers with alkyl ether side groups or with alkoxy or aryloxy side units that bear hydroxyl or carboxylic acid groups are soluble in water and are nonbiodegradable (structures 16.13 in Table 16.1). The most heavily investigated are the MEEP-type systems (structure 16.13a in Table 16.1) with methoxyethoxyethoxy or similar side chains. Like PEO and HEMA, they can be crosslinked to form hydrogels that absorb water below a critical solution temperature (Tc) but extrude the water above this temperature. Devices are being developed that use this phenomenon to deliver drugs through thermally or pH-responsive membranes.
(14) Poly(acrylic acid).
This is a nonbiodegradable polymer (structure 16.14 in Table 16.1) that is soluble only in basic aqueous media. Its main biomedical use is in dental restoration resins in combination with silicates. In solution it is a polyelectrolyte and is capable of triggering a strong antigenic (i.e., rejection) response by the body’s immunological system.
b. Bioerodible Materials
(1) Collagen.
The idea that materials derived from living things might be effective biomaterials has an intuitive appeal. Heart valves from pigs (porcine valves) have been implanted routinely and successfully in humans. However, there are limits to the use of biologically derived materials. The human body has a sophisticated surveillance and rejection system that prevents widespread use of donor human or animal tissues. Moreover, the transmission of bacterial or viral infections is an ever-present possibility.
Collagen is a protein that is present in a wide gamut of mammalian tissues ranging from skin and internal organs to bone. The protein is a copolymer that consists mainly of 80–85% glycine residues. It can be processed by hydrolysis to break crosslinks and reduce the molecular weight, and can be regenerated in different shapes and recrosslinked if necessary. Collagen has been employed for many years in the form of “catgut” in surgical suture fibers for the closing of wounds. Its main disadvantage is that it does not erode quickly in the body. Hence, the sutures must be removed by a second operation. Hydrolyzed collagen is “gelatin” used in food products. Solutions of low-molecular-weight collagen are used as adhesives for repairing perforations in the eardrum.
(2) Alginates.
These are water-soluble, bioerodible, mucopolysaccharides extracted from seaweed and kelp. Alginates (structure 16.16 in Table 16.1) are used as thickening agents in the food and healthcare industries, and they have a long history of safe biocompatibility. Because alginates can be crosslinked ionically by calcium ions, they have been studied for conversion into microspheres for controlled drug delivery.
(3) Poly(lactic-glycolic acid) (PLGA).
Of all the available bioerodible medical materials, PLGA (structure 16.17 in Table 16.1) has received by far the most attention. This polymer was developed commercially in the mid-1960s initially as a bioerodible surgical suture material. There are several versions of PLGA, with different ratios of the two monomer residues and various molecular weights. Synthesis is via the ring-opening polymerization of the “glycolide” cyclic dimers of glycolic and lactic acids. Poly(glycolic acid) hydrolyzes faster than does poly(lactic acid), presumably because it lacks the protective methyl group at the α position. Thus, different co-monomer ratios yield polymers with different rates of hydrolysis. The ultimate hydrolysis products are glycolic and lactic acids. Both are species normally present in the body, but both are acidic. Thus, tissue healing around a suture or implant may be inhibited by the low pH. PLGA is now used in experimental drug delivery devices, bone cements, microspheres, tissue engineering scaffolds, and many other applications. It has FDA approval for certain uses.
In addition to the monomer ratios in the polymer, the speed of erosion depends on the surface area exposed to the aqueous medium (smaller objects erode faster), the degree of polymer crystallinity (crystallinity slows hydrolysis), and the shape of the object. For example, because the surface area: volume ratio of a sphere increases as a sphere contracts in size, spherical devices may not release a drug in a “zero-order” (i.e., constant-release-rate) manner. Note that for drug delivery applications and for uses where strength must be retained even as hydrolysis proceeds, the erosion should take place at the surface and not within the bulk material. Bulk erosion could lead to the catastrophic release of a drug or premature failure of a suture. However, for tissue engineering applications, bulk erosion may be needed to promote uniform cell replication and colonization.
The main defects of PLGA are the limited range of hydrolysis rates and the acidity of the hydrolysis products (reaction 1). The acidity factor is more of a problem for tissue engineering than for drug delivery or surgical devices.
(4) Polyanhydrides.
Until relatively recently, polyanhydrides (structure 16.18 in Table 16.1) were an orphan of the synthetic polymer world because of their hydrolytic sensitivity. They are formed by condensation reactions of dicarboxylic acids, and they hydrolyze back to those acids in contact with water. However, the sensitivity to water depends on whether the polymer backbone contains aliphatic or aromatic residues; the latter have a slowing effect on the hydrolysis rate. Intermediate hydrolysis rates are possible by using both aliphatic and aromatic residues in the same polymer. Polyanhydrides have been used for the localized delivery of a powerful antitumor agent in the treatment of an aggressive brain cancer.
(5) Polycaprolactone and Poly(trimethylene carbonate).
These are two polymers that are receiving increased attention as bioerodible materials. Polycaprolactone (structure 16.19 in Table 16.1) hydrolyzes at a slower rate than does PLGA to give HO(CH2)5C(O)OH The elastomeric polycarbonate (structure 16.20 in Table 16.1) is also used to slow the rate of hydrolysis or other bioerodible polymers, particularly in block copolymer structures.
(6) Bioerodible Polyphosphazenes.
The types of side groups connected to a poly-phosphazene chain govern the stability of the polymer to aqueous media and thus its capacity for bioerosion (see structure 16.21 in Table 16.1). Side groups such as amino acid esters, glucosyl or glyceryl units, and imidazole groups sensitize the polymer to hydrolysis. Those polymers with ethyl glycinate or ethyl alanate side groups have been studied in the greatest detail. The hydrolysis products are the amino acid, ethanol, phosphate, and ammonia; the latter two products provide a pH-buffered medium. The glycine derivative hydrolyzes faster than does its alanine counterpart, which in turn is more sensitive to hydrolysis than the phenylalanine derivative. Thus, the ratio of the two or three different side groups in a polymer controls the overall erosion rate. Other amino acid ester side groups allow property tuning over an even wider range. These polymers have been investigated for controlled drug delivery and especially for bone regeneration applications (see Section C.5 and C.6).
C. MATERIALS FOR SPECIFIC MEDICAL APPLICATIONS
This section provides a summary of how and why different materials are utilized in specific medical devices. The purpose is to give examples that illustrate major areas of medicine where traditional materials are used and where new materials are needed. The emphasis is on cardiovascular materials, sutures and other surgical devices, drug and vaccine delivery, orthopedic applications, and tissue engineering.
1. Cardiovascular Materials
a. General Features.
A variety of materials are used in the following cardiovascular devices—artificial heart pumps and valves, replacement blood vessels, stents, pacemakers, heart–lung machines, and renal dialysis equipment. In all these devices (except pacemakers), materials come into intimate contact with circulating blood. Blood is a complex fluid that contains proteins, salts, red and white corpuscles, and antibodies dissolved or suspended in water. It is an unstable fluid in the sense that it is capable of coagulating in response to trauma or irritation or the presence of an “unnatural” material. Coagulation, or thrombus formation, follows a chain reaction of molecular responses often preceded by the deposition of proteins on the surface.
Although it was once widely believed that hydrophilic surfaces provide the best protection against the coagulation process, it has been found in practice that hydrophobic surfaces such as those found in poly(tetrafluoroethylene), silicones, fluorinated polyphosphazenes, or polyesters provide the best protection. Explanations range from the role of the surface in attracting anticoagulent proteins like albumin, to the ability of the host’s endothelial cells to colonize a hydrophobic surface and insulate blood from the polymer. Control of surface properties is discussed in Chapter 15.
b. Prosthetic Heart Valves.
These are widely used in cardiovascular surgery. They fall into three categories—porcine valves (from pigs), caged ball valves, and leaflet valves. The leaflet valve group is divided into those with a single flap or those with two flaps in a trapdoor configuration. A caged ball valve and a leaflet valve are shown in Figure 16.2.
Porcine valves (mainly collagen) are obtained from the animal and are then stabilized (crosslinked) with formaldehyde before being sutured into the heart tissue. These valves give rise to the fewest complications, but have shorter lifetimes than do mechanical valves. Caged ball valves consist of a silicone rubber sphere retained in a stainless-steel cage, with the device sutured to the heart tissue through a Dacron© cloth surround. An example was shown in an earlier chapter (Figure 1.3). The ball moves back and forth in the cage in response to fluid flow. Ridging of the silicone sphere due to repeated impact with the cage, perhaps exacerbated by the absorption of lipids, is a long-term problem. These valves have the highest incidence of failures due to blood clotting (17% within 5 years, 44% within 15 years). Bileaflet valves made from pyrolytic carbon have the lowest rate of thromboembolism (13% after 5 years, 35% after 15 years), but are more prone to mechanical failure (by leaflet fracture) than are porcine or caged ball valves. Single-leaflet valves fall in the middle of these ranges of reliability. Porcine valves are as quiet as human valves, caged ball valves are somewhat more noisy, and leaflet valves are the noisiest.
Figure 16.2. (a) A caged ball valve and (b) a leaflet-type prosthetic heart valve (courtesy of Baxter Edwards CVS and Medtronic Heart Valve division).

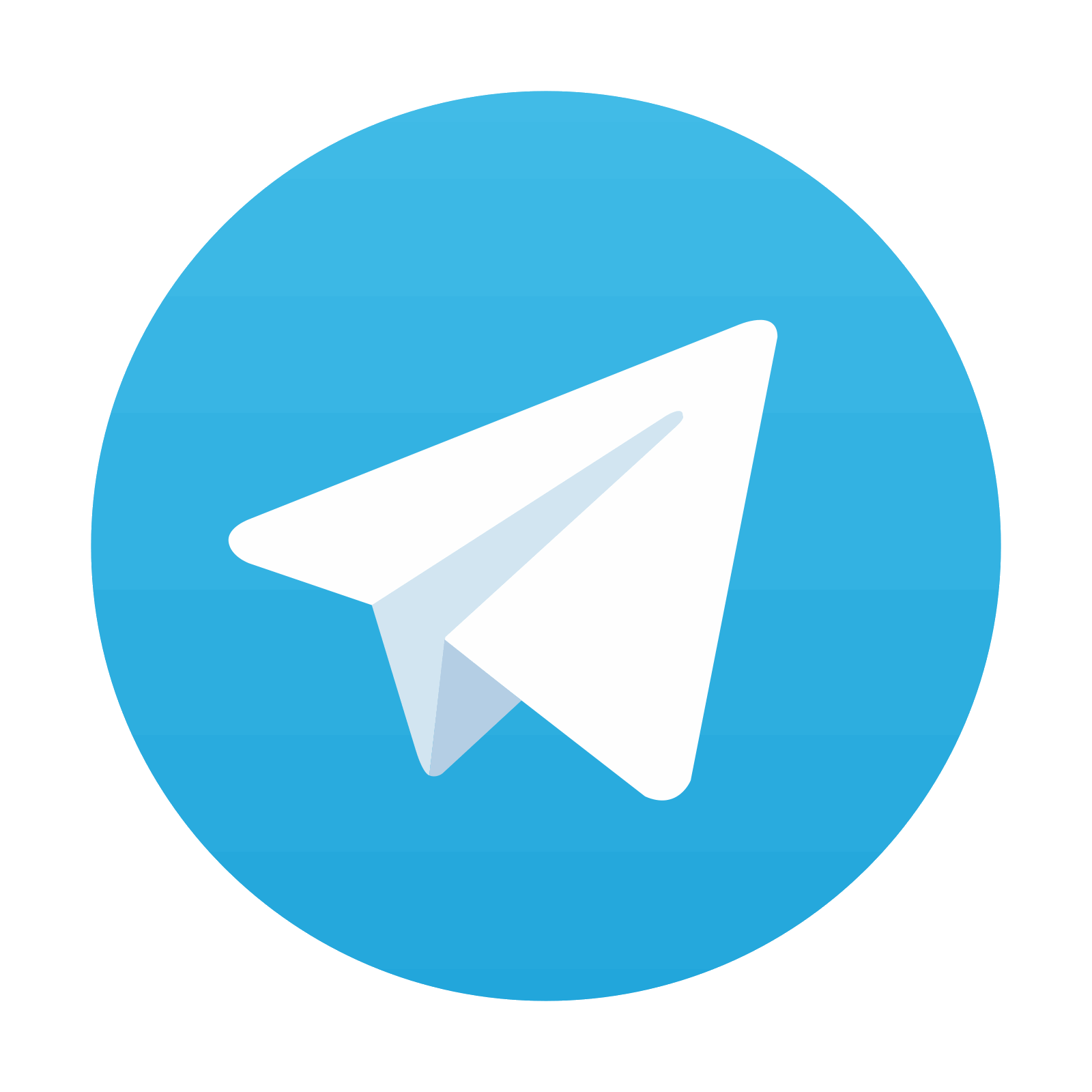
Stay updated, free articles. Join our Telegram channel
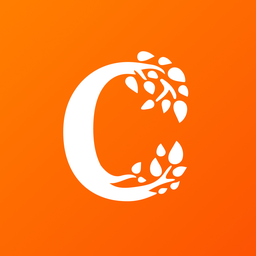
Full access? Get Clinical Tree
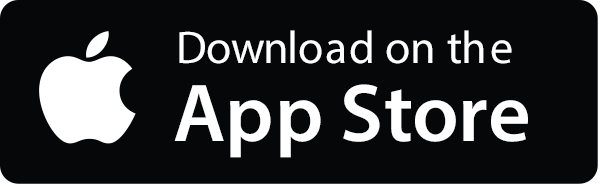
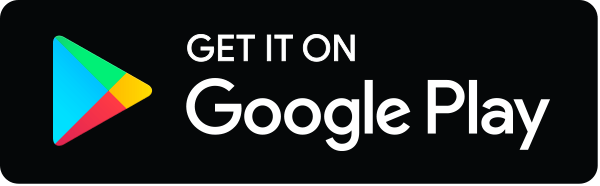