Fig. 6.1
Immobilization of NH2–PEG–NH2 by electrodeposition. The terminated PEGs electrically migrate to and are immobilized on the Ti cathode (a). More terminated amines combine with Ti oxide via an NH–O bond by electrodeposition, while more amines randomly exist as NH3 + in the PEG molecule by immersion (b). An atomic force microscopic image of the PEG-immobilized Ti surface (c)
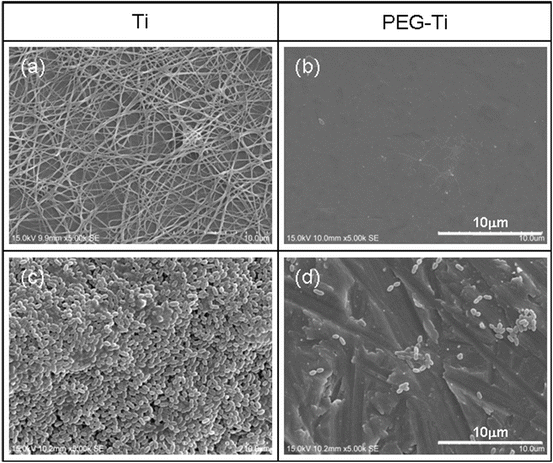
Fig. 6.2
Inhibition of platelet adhesion and biofilm formation on Ti through immobilization of NH2–PEG–NH2 by electrodeposition. (a) Platelet adhesion and fibrin network on untreated Ti, (b) inhibition of platelet adhesion on PEG-immobilized Ti by electrodeposition, (c) biofilm formation on untreated Ti, and (d) inhibition of biofilm formation on PEG-immobilized Ti by electrodeposition
6.3 Immobilization of Biomolecules
6.3.1 Immobilization of Biomolecules
Immobilization of biomolecules is a natural approach to obtain cell and tissue compatibility and biofunctions. It has been found that these coatings act as local mediators of cell adhesion and, in consequence, as a stimulating factor for the growth and proliferation of the cells normally found around the substituted tissue. The tight attachment at the surface of the metallic implant and the conservation of the biological function of the proteins involved are prerequisites for obtaining these highly desirable properties. On the other hand, organic coatings have been scarcely used on metallic implants, because manufacturers hesitate to commercialize the biomolecules immobilized by metallic implant due to the problems on storage, safety, sterilization, etc.
The immobilization of biomolecules on the metallic surface can be achieved using self-assembled monolayers as cross-linkers. Self-assembled monolayers provide chemically and structurally well-defined surfaces that can often be manipulated using standard synthetic methodologies [13]. Thiol on self-assembled monolayers [14, 15] and siloxane-anchored self-assembled monolayers [16] has been particularly well studied. A problem related to the application of immobilized biomolecules via silanization techniques is the hydrolysis of siloxane films when exposed to aqueous (physiological) conditions [17]. More recently, alkyl phosphate films that retain robust under physiological conditions [18] have been used to provide an ordered monolayer on tantalum oxide surfaces [19, 20], and alkalphosphonic acids have been used to coat the native oxide surfaces of metals and their alloys inducing iron [21], steel [22], and Ti [23].
6.3.2 Peptide
In a living tissue, the most important role played by the ECM has been highlighted to favor cell adhesion [24]. Studies have shown that interactions occur between cell membrane receptors and adhesion proteins derived from the bone matrix, such as type I collagen or fibronectin [25]. These proteins are characterized by a RGD (Arg-Gly-Asp) motif which specially transmembrane connections between the actin cytoskeleton and the RGD motif, and the whole system can activate several intracellular signaling pathways modulating cell behavior (e.g., proliferation, apoptosis, shape, mobility, gene expression, and differentiation) [26].
Due to the main role of the RGD sequence in cell adhesion, several research groups have developed biofunctionalized surfaces by immobilization of RGD peptides. Grafting RGD peptides has been performed on different biomaterials, such as Ti [27–29], and has been shown to improve osteoconduction in vitro. Methodologies differ by the conformation of RGD and by the technique used for peptide immobilization [24, 25, 28–30]. Since the graft of an RGD peptide is known to be efficient in bone reconstruction [31], the challenge is to develop simple and cheap methods to favor cell anchorage on biomaterial surfaces [29, 30]. Self-assembled molecular monolayers bearing RGD moieties have been grafted to numerous surfaces, using either silanes [32], phosphonates on oxidized surfaces [29], or thiols on Au [30], but have encountered some application problems for large-scale production. Phosphonates are known to adsorb on Ti. To be mechanically and physiologically stable, phosphonate layers have to be covalently bound to the material surface using drastic conditions [33, 34] which are not compatible with biomolecule stability. Monolayers of RGD phosphonates have been achieved using a complex multistep process which necessitates to tether a primer onto Ti surface, then a linker, and finally the peptide [35]. To immobilize RGD to the electrodeposited PEG on Ti, PEG with an -NH2 group and a -COOH group (NH2–PEG–COOH) must be employed. One terminal group, −NH2, is required to bind stably with a surface oxide on a metal. On the other hand, the other terminal group, −COOH, is useful to bind biofunctional molecules such as RGD as shown in Fig. 6.3 [36]. This RGD/PEG/Ti surface accelerates the calcification by MC3T3-E1 cell [37]. The calcification level is the highest on the RGD/PEG/Ti surface (Fig. 6.4), and the bone formation on the RGD/PEG/Ti surface is accelerated compared to RGD/Ti surface in rabbit [38].
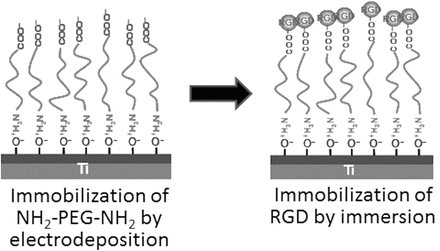
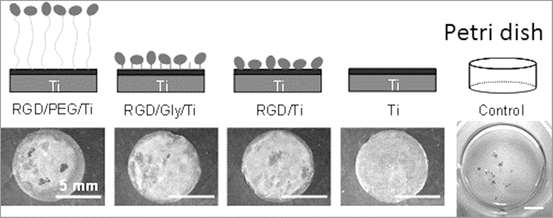
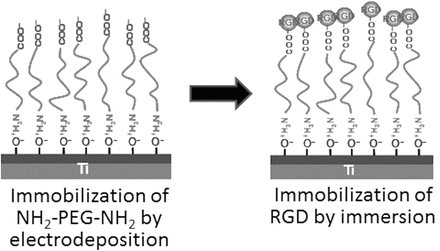
Fig. 6.3
Immobilization of RGD peptide on Ti through electrodeposited NH2–PEG–COOH
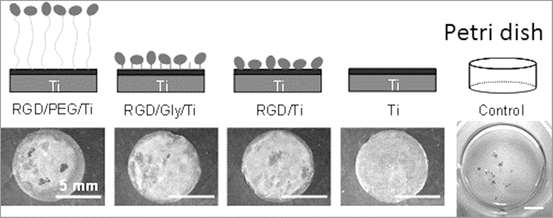
Fig. 6.4
Immobilization mode of RGD peptide on Ti and calcification by MC3T3-E1 cell on each specimen
Glycine (G)–arginine (R)–glycine (G)–asparaginic acid (D)–serine (S) sequence peptide (GRGDS peptide) is coated through the chloride activation technique to enhance adhesion and migration of osteoblastic cells [39]. The expression levels of many genes in MC3T3-E1 cells are altered.
6.3.3 Proteins and Collagen
Among the relevant molecules involved in biochemical modification of bone-contacting surfaces, growth factor, such as bone morphogenetic protein-2 (BMP-2), is of primary interest. BMP-2 has been known to play an important role in bone healing processes and to enhance therapeutic efficiency. Ectopic bone formation by BMP-2 in animals has been well established following the first reports of BMP-2 by the Urist research group [40–42]. Synthetic receptor binding motif mimicking BMP-2 is covalently linked to Ti surfaces through a chemical conjunction process [43]. A complete and homogeneous peptide overlayer on the Ti surfaces; the content is further measured by gamma counting. Biological evaluations show that the biochemically modified Ti is active in terms of cell attachment behavior. Ti surfaces can enhance the rate of bone healing as compared with untreated Ti surface. Bone morphogenetic protein-4 (BMP-4) is immobilized on a Ti–6Al–4V alloy through lysozyme to improve the hard tissue response [44]. Proteins are silane-coupled to the oxidized surfaces of the Co–Cr–Mo alloy, the Ti–6Al–4V alloy, Ti, and the Ni–Ti alloy to improve tissue compatibility [45].
Biomolecules are also used to accelerate bone formation and soft tissue adhesion on a material. Type I collagen is immobilized by immersion in the collagen solution [46]. Type I collagen production increases with modification by ethane-1,1,2-triphosphonic acid and methylenediphosphonic acid grafted onto Ti [47]. Type I collagen is grafted with glutaraldehyde as a cross-linking agent [48]. For the electrodeposition, it is found that an alternating current (AC) between −1 and +1 V vs. SCE with 1 Hz is more effective than direct current (DC) to immobilize type I collagen to Ti and durability in water is high [49] as shown in Fig. 6.5.
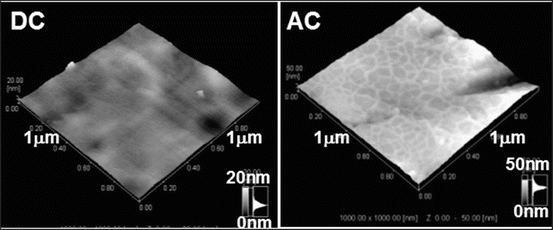
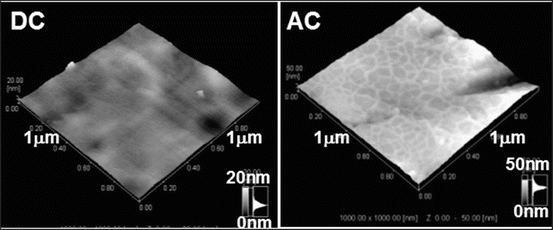
Fig. 6.5
Atomic force microscopic images of Ti surfaces with electrodeposited collagen using DC potential or AC potential
6.3.4 Hydrogel and Gelatin
Immobilization or coating of hydrogel to metal surface is currently being attempted to add a drug delivery ability to orthopedic implant and stents or fluorescent sensing ability to microchips. Currently, synthetic polymeric hydrogels like poly-(hydroxyethylmethacrylate) (pHEMA) and poly(hydroxyethylacrylate) (pHEA) are widely used as compliant materials particularly in the case of contact with blood or other biological fluids [52]. Despite having good flexibility in the swollen state, hydrogels usually lack suitable mechanical properties, and this could greatly impair their use as coating materials for surgical procedure. Moreover, in case of inadequate adhesion between the hydrogel coating and the metal surface, a breakage at the coating–steel interface might occur [53]. A spray-coated method has been set up with the aim to control the coating of pHEMA onto the complex surface of a 316L steel stent for percutaneous coronary intervention (PCI) [54]. The pHEMA coating evaluation of roughness wettability together with its morphological and chemical stability after three cycles of expansion–crimping along with preliminary results after 6 months demonstrates the suitability of the coating for surgical implantation of stent.
An alternative and very promising synthetic route is represented by electrochemical polymerization, which leads to direct thin film coatings on the metal substrates with interesting applications either for corrosion protection or for the development of bioactive films [55–58]. As far as orthopedic field is concerned, in recent years, many procedures based on surface modification have been suggested to improve the biocompatibility and biofunctionality of Ti-based implant [59]. 2-hydroxy-ethyl-methacrylate (HEMA), a macromer poly(ethylene-glycol diacrylate) (PEGDE), and PEGDE copolymerized with acrylic acid were used to obtain hydrogels. A model protein and a model drug were entrapped in the hydrogel and released according to pH change [60].
6.4 Other Polymer Coatings
6.4.1 Bonding of Polymers with Metals Through Silane-Coupling Agent
The interfacial chemical structure governing the bonding strength, especially at the nanometer level, is one of the most challenging aspects to the development of composite materials. The combination of a Ti alloy with a resin for crown facings has been attempted [61]. In particular, silane-coupling agents containing S–H groups and Si–O–CH3 groups are widely used to combine dental alloys with resins [62]. The S–H group works as a bonding agent with polymers, while the Si–O–CH3 group works as a bonding agent with metals. The mechanical properties and durability of composite resin increase with the silanized filler [63–66]. However, in most studies on materials using silane-coupling agents in the field of dentistry, only the bonding strength is evaluated and discussed, and there are few reports that examine and discuss the chemical structures at the bonding interface and how they influence the bonding strength.
Studies on silane-coupling agents to combine polymers with metals have been performed in other fields. An aluminum–vegetable oil composite using a silane-coupling agent has been developed [67, 68]. Rubber-to-metal bonding by a silane-coupling agent was investigated [69]. In addition, the surface modification of stainless steel by grafting poly(ethylene glycol) using a silane-coupling agent has been reported [6]. However, only the chemical structure is investigated in these studies. In other words, the relationship between the bonding strength and the interfacial chemical structure containing a silane-coupling agent layer has not been studied.
The unequivocal relationship between the shear bonding strength and the chemical structure at the bonding interface of a Ti–SPU composite through a silane-coupling agent (γ-mercaptopropyl trimethoxysilane (γ-MPS)) is investigated [70]. The bonding interface between SPU and Ti substrate is determined by the thickness of γ-MPS layer as shown in Fig. 6.6. On the other hand, the shear bond strength of the Ti/SPU interface increased with ultraviolet (UV) irradiation according to the increase of the cross-linkage in SPU. Platelet adhesion to Ti is inhibited by SPU, as shown in Fig. 6.7. This technique is used for the creation of a new meta-based material having high strength, high toughness, and biofunction. UV irradiation to a Ti–SPU composite is clearly a factor governing the shear bond strength of the Ti/SPU interface [71]. In addition, active hydroxyl groups on the surface oxide film are clearly factors governing the shear bond strength [72]. After good bonding between metal and polymer is produced, biofunctionalization techniques developed in the field of polymers could be applied to the composite materials.
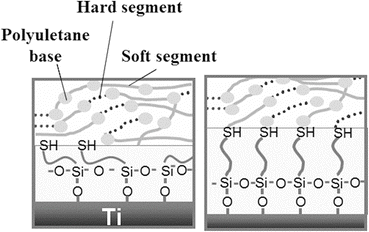
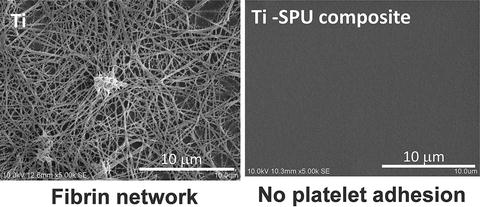
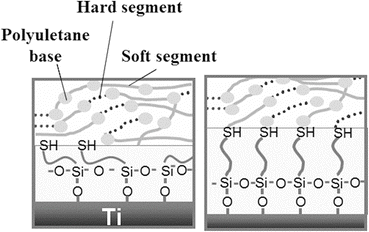
Fig. 6.6
Difference in bonding interface structure between thin (left) and thick (right) γ-MPS layers
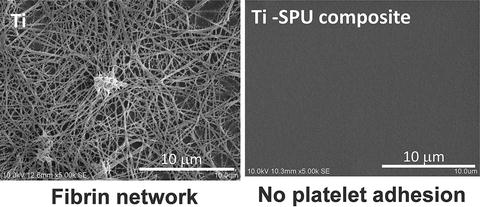
Fig. 6.7
Inhibition of platelet adhesion and fibrin network formation on SPU-coated Ti
The effects of different kinds of terminal functional groups and the thickness of the silane layers (SIL) on the adhesive strength between Ti–29Nb–13Ta–4.6Zr (TNTZ) alloy and SPU were investigated by means of shear bonding tests. The following silane-coupling agents were employed in this study: 3-methacryloxypropyltrimethoxysilane (γ-MPTS), aminopropyltriethoxysilane (APS), and γ-MPS. Furthermore, the shear bonding strength of the TNTZ/SIL/SPU interface was also characterized after immersion in water for 30 days. Silane-coupling treatment produces a tenfold increase in the shear bonding strength, independent of the type of terminal functional groups and the thickness of the silane layers. The TNTZ/SIL/SPU composites are partially fractured at the interfaces of the TNTZ/SIL, while the rest of the fracture occurs at the interfaces of the SIL/SPU in single sample. The shear bonding strength decreases after immersion in water for 30 days when APS and γ-MPS are used as the silane-coupling agents, because stable chemical bonding is not achieved between the silane layer and SPU, whereas the bonding of the γ-MPTS composite is not affected by exposure to water [73]. The number of hydroxyl groups increases with an increase in treatment time at a H2O2 concentration of 5 %. On the other hand, an increase from 5 to 30 % in H2O2 concentration leads to a decrease in the number of hydroxyl groups on the TNTZ surface, because at higher H2O2 concentrations, the reaction that consumes the hydroxyl groups is dominant. The shear bonding strength is doubled compared with the untreated TNTZ/SIL/SPU interface. Although the shear bonding strength decreases after immersion in water for 30 days when APS and γ-MPS are used, TNTZ/γ-MPTS/SPU composites exhibit good durability to water and maintain an equivalent shear bonding strength before immersion in water [74]. The adhesive strength of the SPU coating on the nanotube structure formed on TNTZ by anodization for 3,600 s increases by 50 %. These improvements in the adhesive strength of SPU are the result of an anchor effect introduced by the nanostructures formed by anodization. Fracture occurs at the interface of the nanoporous structure and the SPU coating layer. In contrast, in the case that SPU coating has been performed on the nanotube structure, fracture occurs inside the nanotubes [75].
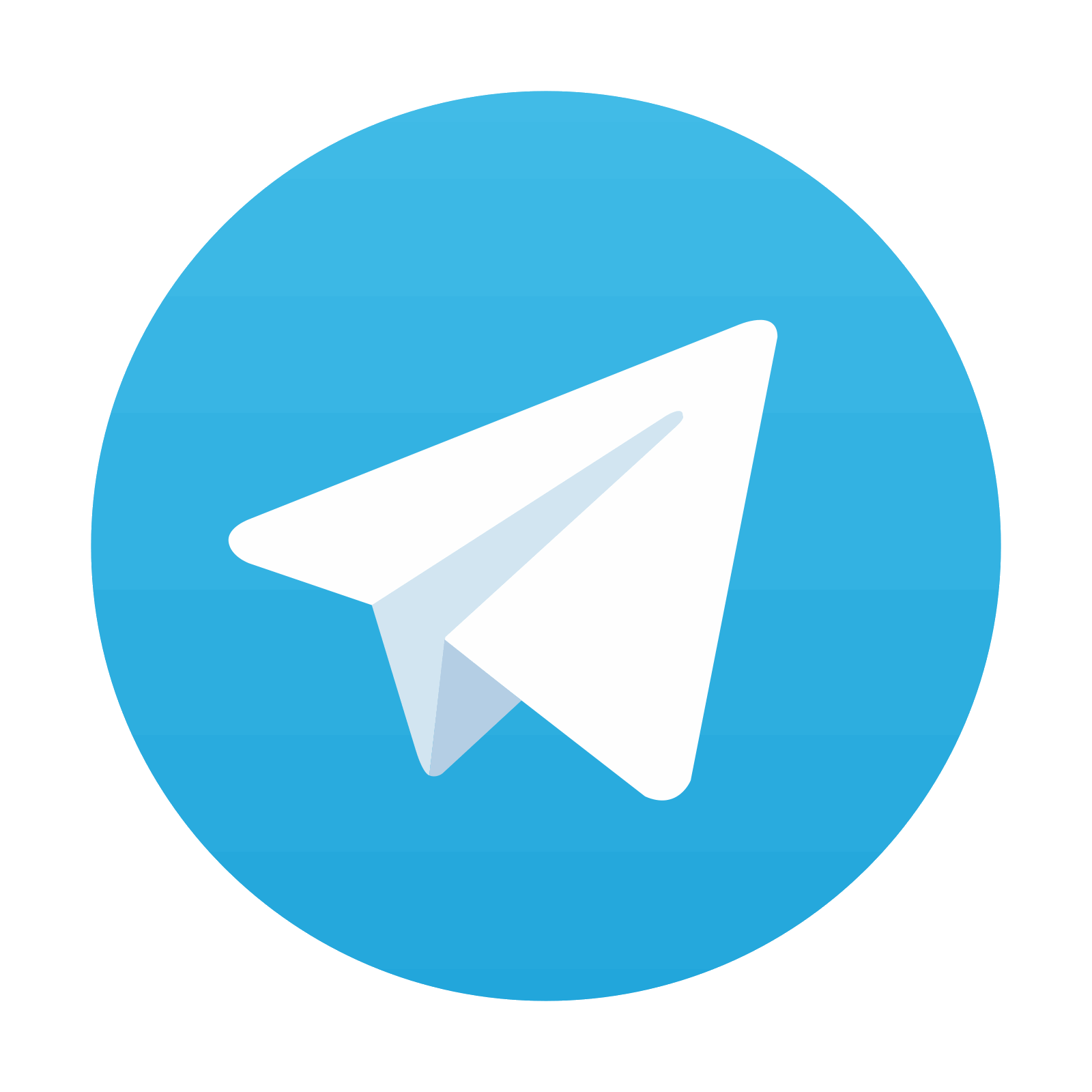
Stay updated, free articles. Join our Telegram channel
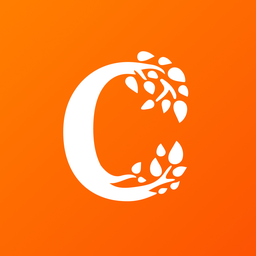
Full access? Get Clinical Tree
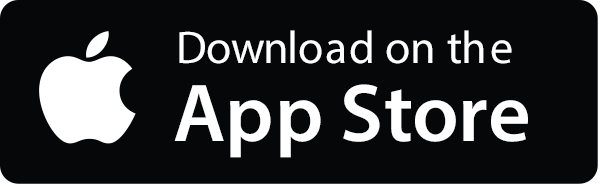
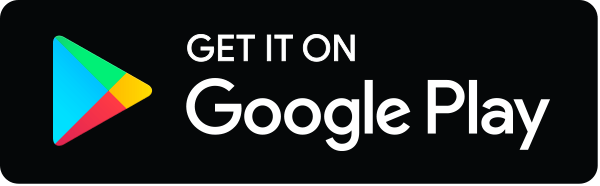