C. An antagonist is a drug that binds to the receptor without activating the receptor (Fig. 2-2).
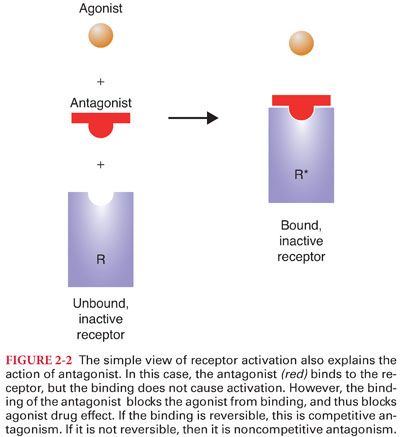
1. Antagonists block the action of agonists simply by getting in the way of the agonist, preventing the agonist from binding to the receptor and producing the drug effect.
2. Competitive antagonism is present when increasing concentrations of the antagonist progressively inhibit the response to the agonist.
3. Noncompetitive antagonism is present when, after administration of an antagonist, even high concentrations of agonist cannot completely overcome the antagonism.
D. Although this simple view of activated and inactivated receptors explains agonists and antagonists, it has a more difficult time with partial agonists and inverse agonists. It turns out that receptors have many natural conformations, and they naturally fluctuate between these different conformations (Figs. 2-3 and 2-4).
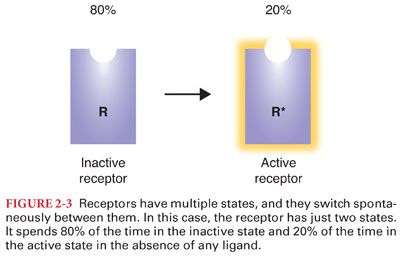
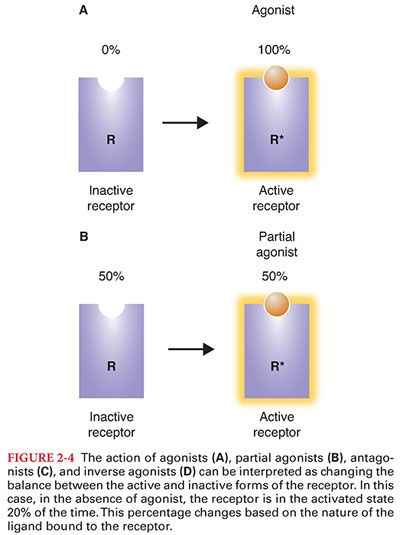
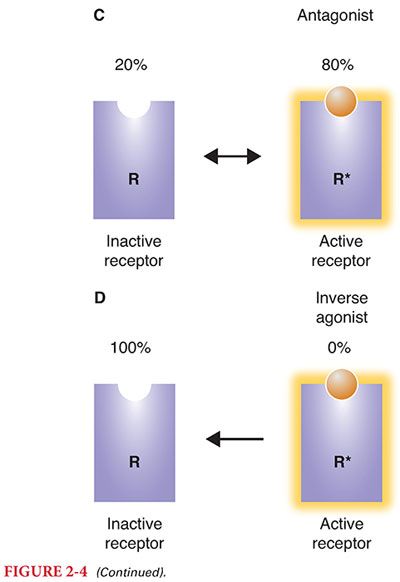
II. Receptor Action
A. The number of receptors in cell membranes is dynamic and increases (upregulates) or decreases (downregulates) in response to specific stimuli.
B. Changing receptor numbers is one of many mechanisms that contribute to variability in response to drugs.
III. Receptor Types
A. Many of the receptors thought to be the most critical for anesthetic interaction are located in the lipid bilayer of cell membranes (opioids, intravenous sedative hypnotics, benzodiazepines, beta blockers, catecholamines, muscle relaxants) and interact with membrane bound receptors.
B. Other receptors are intracellular proteins and interact with insulin and steroids.
C. Proteins function in the body as small machines, catalyzing enzymatic reactions and acting as ion channels.
1. When a drug binds to a receptor, it changes the activity of the machine, typically by enhancing its activity (propofol increases the sensitivity of the γ-aminobutyric acid receptor [GABAA] to GABA, the endogenous ligand), decreasing its activity (ketamine decreases the activity of the N-methyl-D-aspartate [NMDA] receptor), or triggering a chain reaction (opioid binding to the μ opioid receptor activates an inhibitory G protein that decreases adenylyl cyclase activity).
2. The protein’s response to binding of the drug is responsible for the drug effect.
IV. Pharmacokinetics is the quantitative study of the absorption, distribution, metabolism, and excretion of injected and inhaled drugs and their metabolites (what the body does to a drug).
A. Pharmacokinetics determines the concentration of a drug in the plasma or at the site of drug effect.
B. Pharmacokinetic variability is a significant component of patient-to-patient variability in drug response and may result from genetic modifications in metabolism; interactions with other drugs; or disease in the liver, kidneys, or other organs of metabolism.
C. Absorption, metabolism, distribution, and elimination are processes that are fundamental to all drugs (can be described in basic physiologic terms or using mathematical models).
1. Physiology can be used to predict how changes in organ function will affect the disposition of drugs.
2. Mathematical models can be used to calculate the concentration of drug in the blood or tissue following any arbitrary dose, at any arbitrary time.
V. Distribution
A. When drugs are administered, they mix with body tissues and are immediately diluted from the concentrated injectate in the syringe to the more dilute concentration measured in the plasma or tissue. This initial distribution (within 1 minute) after bolus injection is considered mixing within the “central compartment” (Fig. 2-5).
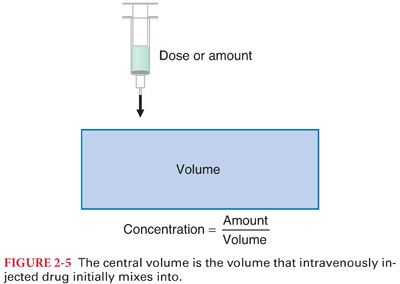
B. It may take hours or even days for the drug to fully mix with all bodily tissues because some tissues have very low perfusion.
C. Many anesthetic drugs are highly fat soluble and poorly soluble in water.
1. High fat solubility means that the molecule will have a large volume of distribution because it will be preferentially taken up by fat, diluting the concentration in the plasma.
2. The extreme example of this is propofol, which is almost inseparable from fat.
D. Following bolus injection, drug primarily goes to the tissues that receive the bulk of arterial blood flow: the brain, heart, kidneys, and liver. These tissues are often called the vessel rich group.
1. The rapid blood flow ensures that the concentration in these highly perfused tissues rises rapidly to equilibrate with arterial blood.
2. For highly fat-soluble drugs, the capacity of the fat to hold the drug greatly exceeds the capacity of highly perfused tissues.
a. With time (initially, the fat compartment is almost invisible because fat blood flow is low), the fat gradually absorbs more and more drug, sequestering it away from the highly perfused tissues.
b. This redistribution of drug from the highly perfused tissue to the fat accounts for a substantial part of the offset of drug effect following a bolus of an intravenous anesthetic or fat-soluble opioid (fentanyl).
VI. Protein Binding
A. Most drugs are bound to some extent to plasma proteins, primarily albumin (acidic drugs) and α1-acid glycoprotein and lipoproteins (basic drugs).
B. Protein binding effects both the distribution of drugs (only the free or unbound fraction is readily cross cell membranes) and the apparent potency of drugs (it is the free fraction that determines the concentration of bound drug on the receptor).
1. The extent of protein binding parallels the lipid solubility of the drug.
2. For intravenous anesthetic drugs, the number of available protein binding sites in the plasma vastly exceeds the number of sites actually bound. As a result, the fraction bound is not dependent on the concentration of the anesthetic and only dependent on the protein concentration.
3. Age, hepatic disease, renal failure, and pregnancy can all result in decreased plasma protein concentration.
a. Alterations in protein binding are important only for drugs that are highly protein bound (>90%).
b. For such drugs, the free fraction changes inversely proportionally with a change in protein concentration. If the free fraction is 2% in the normal state, then in a patient with 50% decrease in plasma proteins, the free fraction will increase to 4%, a 100% increase.
C. Theoretically, an increase in free fraction of a drug may increase the pharmacologic effect of the drug, but in practice, it is far from certain that there will be any change in pharmacologic effect at all.
VII. Metabolism converts pharmacologically active, lipid-soluble drugs into water-soluble and usually inactive metabolites; exceptions are metabolism to active compounds as for diazepam and opioids (morphine-6-glucuronide is more potent than morphine; codeine is a prodrug metabolized to morphine).
A. Pathways of Metabolism. The four basic pathways of metabolism are (a) oxidation, (b) reduction, (c) hydrolysis, and (d) conjugation.
1. Phase I reactions include oxidation, reduction, and hydrolysis, which increase the drug’s polarity and prepare it for phase II reactions.
2. Phase II reactions are conjugation reactions that covalently link the drug or metabolites with a highly polar molecule (carbohydrate or an amino acid) that renders the conjugate more water soluble for subsequent excretion.
3. Hepatic microsomal enzymes (hepatic smooth endoplasmic reticulum but also present in kidneys and gastrointestinal tract) are responsible for the metabolism of most drugs.
B. Phase I enzymes responsible for phase I reactions include cytochrome P-450 enzymes (predominantly hepatic microsomal enzymes), noncytochrome P-450 enzymes, and flavin-containing monooxygenase enzymes.
1. The cytochrome P-450 enzyme system is a large family of membrane-bound proteins.
2. Drugs can alter the activity of these enzymes through induction and inhibition (phenobarbital induces microsomal enzymes and thus can render drugs less effective through increased metabolism).
3. Oxidation. Cytochrome P-450 enzymes are crucial for oxidation reactions. Examples of oxidative metabolism of drugs catalyzed by cytochrome P-450 enzymes include hydroxylation, deamination, desulfuration, dealkylation, and dehalogenation.
4. Reduction. Cytochrome P-450 enzymes are also essential for reduction reactions. Under conditions of low oxygen partial pressures, cytochrome P-450 enzymes transfer electrons directly to a substrate such as halothane rather than to oxygen.
5. Conjugation with glucuronic acid involves cytochrome P-450 enzymes. The resulting water-soluble glucuronide conjugates are then excreted in bile and urine.
6. Hydrolysis. Enzymes responsible for hydrolysis of drugs, usually at an ester bond, do not involve the cytochrome P-450 enzyme system. Hydrolysis often occurs outside of the liver (remifentanil, succinylcholine, esmolol, and the ester local anesthetics are cleared in the plasma and tissues via ester hydrolysis).
C. Phase II enzymes include glucuronosyltransferases, glutathione-S-transferases, N-acetyl-transferases, and sulfotransferases.
1. Glucuronidation is an important metabolic pathway for several drugs used during anesthesia, including propofol, morphine (yielding morphine-3-glucuronide and the pharmacologically active morphine-6-glucuronide), and midazolam (yielding the pharmacologically active 1-hydroxymidazolam).
2. Glutathione-S-transferase (GST) enzymes are primarily a defensive system for detoxification and protection against oxidative stress.
VIII. Hepatic Clearance
A. Although the metabolic capacity of the body is large, it is not possible that metabolism is always proportional to drug concentration, because the liver does not have infinite metabolic capacity.
B. The rate at which drug flows out of the liver must be the rate at which drug flows into the liver, minus the rate at which the liver metabolizes drug (Figs. 2-6 and 2-7).
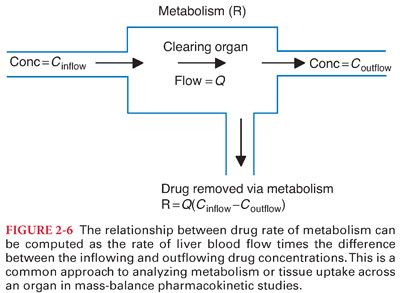
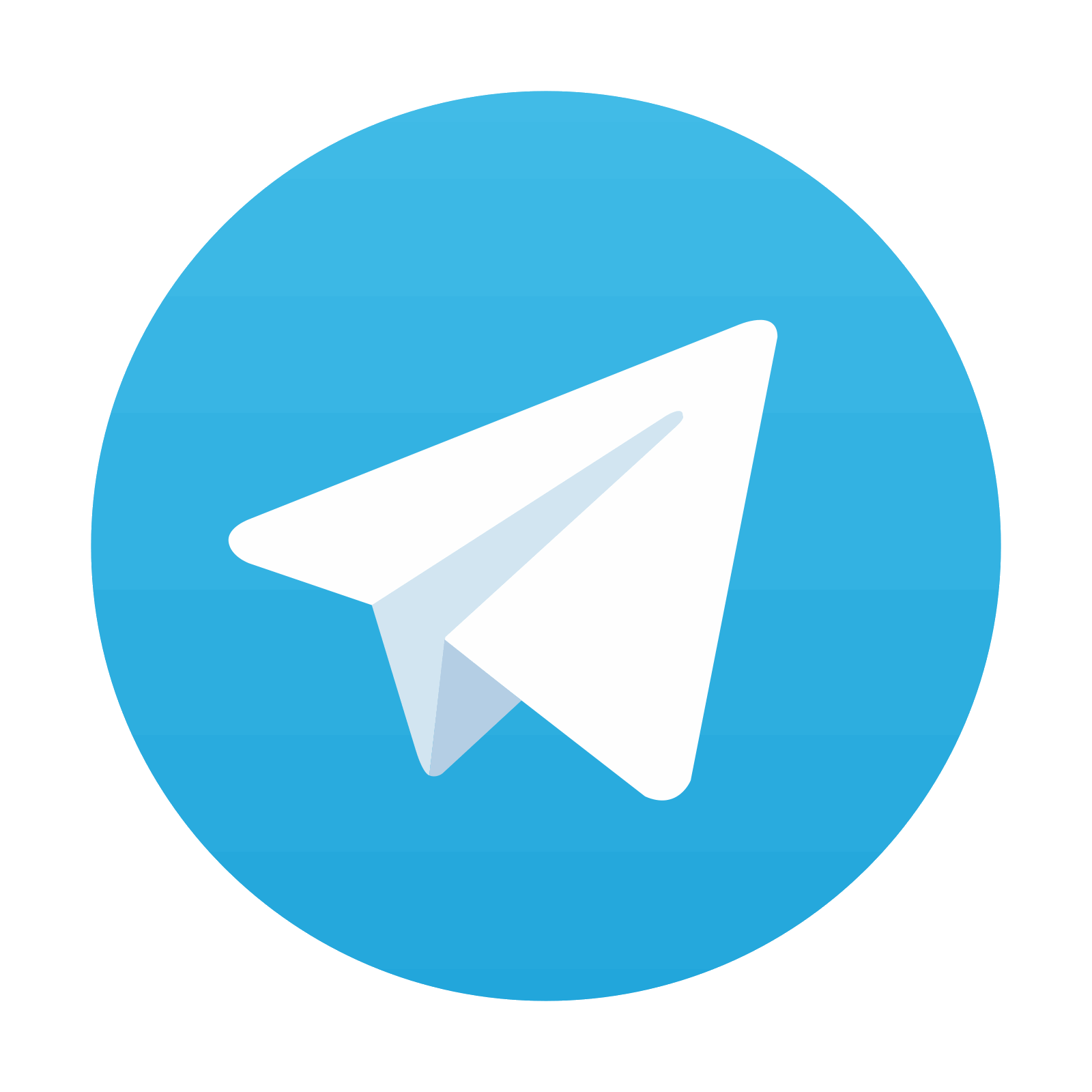
Stay updated, free articles. Join our Telegram channel
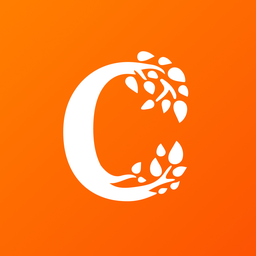
Full access? Get Clinical Tree
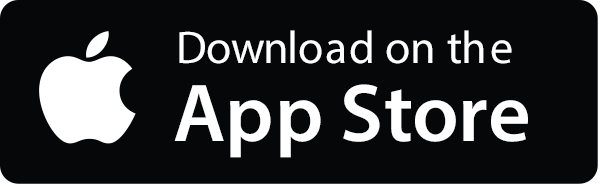
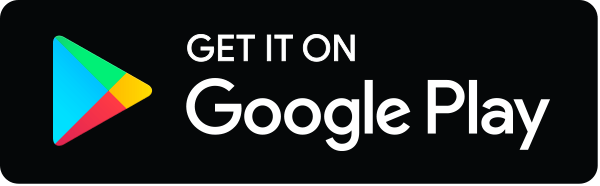