Figure 41.1. Oxygen dissociation curve.
Source: This figure was published in Walker HK, Hall WD, Hurst JW, Hurst HW. Clinical Methods, 3rd ed. 1990:256. Boston: Butterworths. © Elsevier.
Although they are clinically valuable parameters, neither Pao2 nor Sao2 provides quantitative information about the total amount of oxygen in arterial blood. The total quantity of oxygen in arterial blood is referred to as the arterial oxygen content (Cao2) and is described by the equation:
Where Hb is hemoglobin (in g/dL), 1.39 is the volume of oxygen (mL) that can be bound by a gram of hemoglobin, and 0.003 is the solubility coefficient of oxygen in plasma. Cao2 is expressed in milliliters of oxygen per deciliter.
The arterial oxygen content, therefore, is essentially the sum of the Sao2 and Pao2 (corrected for hemoglobin content and solubility, respectively). Cao2 is the most physiologically relevant estimation of the quantity of oxygen delivered to peripheral tissues.
A-a GRADIENT AND THE ALVEOLAR GAS EQUATION
The transfer of oxygen from the alveolus to the blood in the pulmonary capillaries is described by the alveolar (A) to arterial (a) gradient. The A-a gradient provides a rough estimation of the relative ease by which oxygen transfers across the alveolar-capillary membrane. The partial pressures of oxygen in the alveolar and arterial compartments are used to determine the A-a gradient.
The partial pressure of oxygen in arterial blood (the Pao2) is directly measured by obtaining an ABG. The partial pressure of oxygen in the alveoli (Pao2) must be calculated by the alveolar gas equation:
where PIO2 = (Pbar – PH2o) × FIO2, Pbar is barometric pressure (760 mm Hg at sea level), Ph2o is the partial pressure of water vapor (47 mm Hg), and Fio2 is the fraction of inhaled oxygen. Paco2 is the partial pressure of carbon dioxide in arterial blood and R is the respiratory quotient (estimated to be 0.8 under normal conditions).
If the patient is breathing room air (Fio2 = 0.21) at sea level, the equation can be simplified:
A-a gradient = Pao2 (calculated) – Pao2 (measured)
Normal range = 0–10 mm Hg or 2.5 + 0.21 × (age in years) mm Hg.
There are a number of assumptions in the alveolar gas equation that must be considered when using the A-a gradient clinically. The respiratory quotient is the amount of carbon dioxide produced divided by the amount of oxygen consumed, described as:
where is carbon dioxide production and
is oxygen consumption.
The amount of carbon dioxide produced may vary markedly depending on a patient’s metabolic state. For example, patients with sepsis and systemic inflammation may initially have very high CO2 production. Sepsis is also characterized by decreased oxygen uptake, further skewing the respiratory quotient from its assumed value of 0.8.
Fio2 may influence the A-a gradient, as higher Fio2 is associated with a higher A-a gradient. Increasing age is also associated with a higher A-a gradient. These factors need to be considered to determine whether the calculated A-a gradient actually represents abnormal oxygen transfer and uptake.
Hypoxemia can be caused by a variety of pathophysiological processes including ventilation/perfusion () mismatch, shunt, alveolar hypoventilation, reduced partial pressure of oxygen in the inhaled air, or a diffusion abnormality (typically does not cause hypoxemia at rest at sea level). A truly elevated A-a gradient is consistent with
mismatch, shunt, or a diffusion abnormality. In cases of hypoxemia due to alveolar hypoventilation or reduced partial pressure of oxygen, the A-a gradient is normal.
The Pao2/Fio2 ratio is another metric of hypoxemia that is frequently used in the critical care setting. The normal Pao2/Fio2 is 300–500 mm Hg. A Pao2/Fio2 ratio of <300 mm Hg indicates a clinically significant gas exchange derangement.
EFFECTS OF SUPPLEMENTAL OXYGEN
Supplemental oxygen increases the Pao2 in patients who are ventilating normally by increasing the Fio2. Increased Pao2 leads to an increased driving pressure of oxygen across the alveolar membrane. In a patient with mismatch, increased Pao2 leads to an increased Pao2. In the setting of shunt, in which blood passes through the lungs without making contact with the alveolus for gas exchange to occur, supplemental oxygen does not improve hypoxemia.
In the absence of a complete shunt, the amount of increase of Pao2 for a given amount of supplemental oxygen can be estimated. It should be emphasized that measurements of Pao2 vary over time without any significant change in clinical status, so the fidelity of equations predicting changes in Pao2 for a given increase in Fio2 is limited. Additionally, methods of administering oxygen make estimating the precise percentage of oxygen inhaled difficult. Specifically, an awake patient receiving oxygen by nasal cannulae may inhale through his or her mouth, decreasing the relative contribution of the nasally delivered oxygen. Figure 41.2 demonstrates the approximate relationship between increased Fio2 and Pao2 in hypothetical patients with varying degrees of mismatch.
Figure 41.2. Changes in Pao2 with increasing Fio2 with varying degrees of mismatch.
Source: Reprinted with permission from Tobin MJ. Principles and Practice of Mechanical Ventilation. New York: McGraw-Hill; 2006:760.
Plane travel is an interesting circumstance in which supplemental oxygen may be necessary for patients who normally do not require it. The alveolar gas equation reminds us that barometric pressure is the primary driving force of gas into the alveoli. By law, the cabin pressure of planes is set at the equivalent of no higher than 8000 feet (~560 mm Hg); usually cabin pressure is the equivalent of 6000–8000 feet. For people without lung disease or shunt, this decrease in barometric pressure is well tolerated. However, for patients with pulmonary disease, depressurization to 8000 feet may be dangerous. Prior to flying, the predicted Pao2 at 8000 feet should be calculated for such patients using the following equation:
If the patient’s predicted Pao2 is unacceptably low (i.e., <60 mm Hg), providing supplemental oxygen to increase the patient’s Fio2 can counterbalance the effects of decreased barometric pressure while flying. Alternative methods for assessing whether a patient would tolerate plane travel include having the patient breathe hypoxic gas (15.1% oxygen, called the hypoxia altitude simulation test) or measuring Pao2 in a hypobaric chamber. These methods may be more accurate but may also require additional equipment compared with the above equation.
CARBOXYHEMOGLOBINEMIA AND METHEMOGLOBINEMIA
Hemoglobin molecules can lose affinity for oxygen by binding to carbon monoxide or by transforming into methemoglobin. Carbon monoxide avidly and irreversibly binds to hemoglobin, thereby decreasing the binding sites available for oxygen to bind to hemoglobin. Hemoglobin bound to carbon monoxide is referred to as carboxyhemoglobin.
Methemoglobin is formed when ferrous iron (Fe2+) is oxidized to the ferric state (Fe3+); this oxidative change decreases oxygen carrying capacity by changing the hemoglobin tetramer’s ability to bind and release oxygen to tissues. Methemoglobinemia can be congenital or acquired. Congenital forms of methemoglobinemia may be due to enzyme deficiencies (such as diaphorase I deficiency). More commonly, methemoglobin is acquired as a result of oxidizing medications causing ferrous iron to convert to the ferric state. Dapsone, nitrates, local anesthetics (such as benzocaine), trimethoprim, and sulfonamides are potential causes of methemoglobinemia.
In these conditions, an ABG may reveal a normal Pao2. The measured Sao2, however, is abnormal as the amount of oxygen bound to hemoglobin is decreased. It is important to recognize that peripherally measured oxygen saturation levels with pulse oximetry will be normal—an ABG is necessary to determine the true degree of hypoxia in these conditions. Pulse oximetry is normal (or near normal) in these conditions, as light emitted by the pulse oximeter is increased by carboxy- and methemoglobin, which the oximeter reports as oxygen (rather than carbon monoxide saturation or methemoglobin levels).
Both carboxyhemoglobinemia and methemoglobinemia are treated with supplemental oxygen (including intubation and mechanical ventilation if necessary). Methemoglobinemia may be treated with methylene blue (1–2 mg/kg over 5 minutes), as it reduces the iron ion in hemoglobin to its ferrous (Fe2+) state, thereby allowing the hemoglobin to bind and carry oxygen. Carboxyhemoglobinemia may require treatment in a hyperbaric oxygen chamber. Increasing the Pao2 (by increasing the barometric pressure) may make the pressure gradient for oxygen across the alveolar membrane high enough for oxygen to displace avidly bound carbon monoxide from hemoglobin.
ACID–BASE DISORDERS
HYPERCAPNIA AND ACUTE RESPIRATORY ACIDOSIS
Increased carbon dioxide levels (referred to as hypercapnia) occur in the setting of hypoventilation. Carbon dioxide readily diffuses from the bloodstream across the alveolar-capillary membrane; it is removed from alveoli by the process of ventilation. The limiting step in the elimination of carbon dioxide is not diffusion from the pulmonary circulation to the alveoli, rather it is the efficiency and efficacy of ventilation. This relationship is described via the following equation:
where is alveolar ventilation, Vco2 is the metabolic production of CO2, k is a proportionality constant, and Paco2 is the partial pressure of carbon dioxide in arterial blood (in mm Hg).
When alveolar ventilation ( ) decreases, Paco2 must increase proportionally, assuming that
does not change. Understanding that hypoventilation is the primary cause of elevated blood levels of carbon dioxide is important in diagnosing and treating hypercapnia.
Alveolar ventilation cannot be readily measured in the clinical setting. However, minute ventilation () is easily determined:
is the portion of the
that does not include dead space ventilation:
where vd/vt is dead space fraction.
The vd/vt may be measured by the ventilator in mechanically ventilated patients. Specifically, expired carbon dioxide is collected and measured over a certain period of time; vd/vt can be calculated via the following relationship:
where Peco2 is the expired CO2.
Clinically, therefore, the relationship between and Paco2 can be represented by the following equation:
, vd/vt, and Paco2 are all easily measured parameters and
× k is thought to be relatively constant. When
decreases, Paco2 must increase. When vd/vt increases, Paco2 must increase. Both of these situations (increased vd/vt and decreased
) result in alveolar hypoventilation (decreased
).
Acute respiratory acidosis occurs when decreases suddenly. As the continued, efficient elimination of carbon dioxide is important for preservation of a neutral acid–base status, a sudden decrease in
and a sudden increase in Paco2 causes a rapid decrease in pH.
Causes of decreased are classically divided into central and peripheral processes. Central hypoventilation may occur for a variety of reasons. Intoxication, particularly with opiates or other central nervous system (CNS) depressants, may result in a blunted central respiratory drive and decreased
.
Peripheral causes of decreased include neuromuscular disorders or neuromuscular blocking medications that cause weakened or inefficient muscles of respiration. When tidal volume and/or respiratory rate decreases,
decreases, and Paco2 rises. Severe airways obstruction, such as a chronic obstructive pulmonary disease (COPD) exacerbation, may also result in acute hypoventilation and hypercapnia.
It is rare that small increases in vd/vt alone result in hypercapnia. Carbon dioxide so readily diffuses across the alveolar membrane that minimal increases in can compensate for small increases in vd/vt and maintain a normal or near-normal CO2.
Treatment of hypercapnia is centered on treatment of the underlying process. In certain circumstances, mechanical ventilation may be necessary to achieve adequate and
to correct hypercapnia.
COMPENSATORY MECHANISMS
CHRONIC RESPIRATORY ACIDOSIS
Chronic respiratory acidosis is an interesting condition in which a patient experiences persistent alveolar hypoventilation. Persistent alveolar hypoventilation and persistently elevated Paco2 result in changes in kidney function in an effort to restore acid–base neutrality. Specifically, normally functioning kidneys respond to persistent acidemia by increasing retention of bicarbonate while increasing excretion of hydrogen ion. Over several days, the kidneys’ response results in elevated serum bicarbonate levels and the return of serum pH toward normal.
Reasons for chronic alveolar hypoventilation, and thereby chronic respiratory acidosis, include central and peripheral processes (box 41.1). Central processes include pathologies such as obesity hypoventilation syndrome (OHS, or the so-called Pickwickian syndrome.) In patients with OHS, chronic hypoventilation leads to a “reset” of the carotid bodies and the central medullary respiratory centers. The physiologically acceptable Paco2 is liberalized, and centrally mediated respiratory drive is relatively decreased due to increased tolerance for elevated Paco2 by the central respiratory centers. Similarly and rarely, a stroke in the medullary respiratory center can result in central hypoventilation.
Box 41.1 CAUSES OF RESPIRATORY ACIDOSIS.
Chronic obstructive pulmonary disease: Emphysema, severe asthma, chronic bronchitis
Neuromuscular diseases: Amyotrophic lateral sclerosis, diaphragm dysfunction and paralysis, Guillain-Barré syndrome, myasthenia gravis, muscular dystrophy
Chest wall disorders: Severe kyphoscoliosis; status post-thoracoplasty; flail chest; less commonly, ankylosing spondylitis, pectus excavatum, or pectus carinatum
Obstructive sleep apnea
Obesity-hypoventilation syndrome
CNS depression: Drugs (e.g., narcotics, barbiturates, benzodiazepines, other CNS depressants), neurologic disorders (e.g., encephalitis, brainstem disease, trauma), primary alveolar hypoventilation
Other lung and airway diseases: Laryngeal and tracheal stenosis
Lung-protective ventilation in acute respiratory distress syndrome (ARDS)
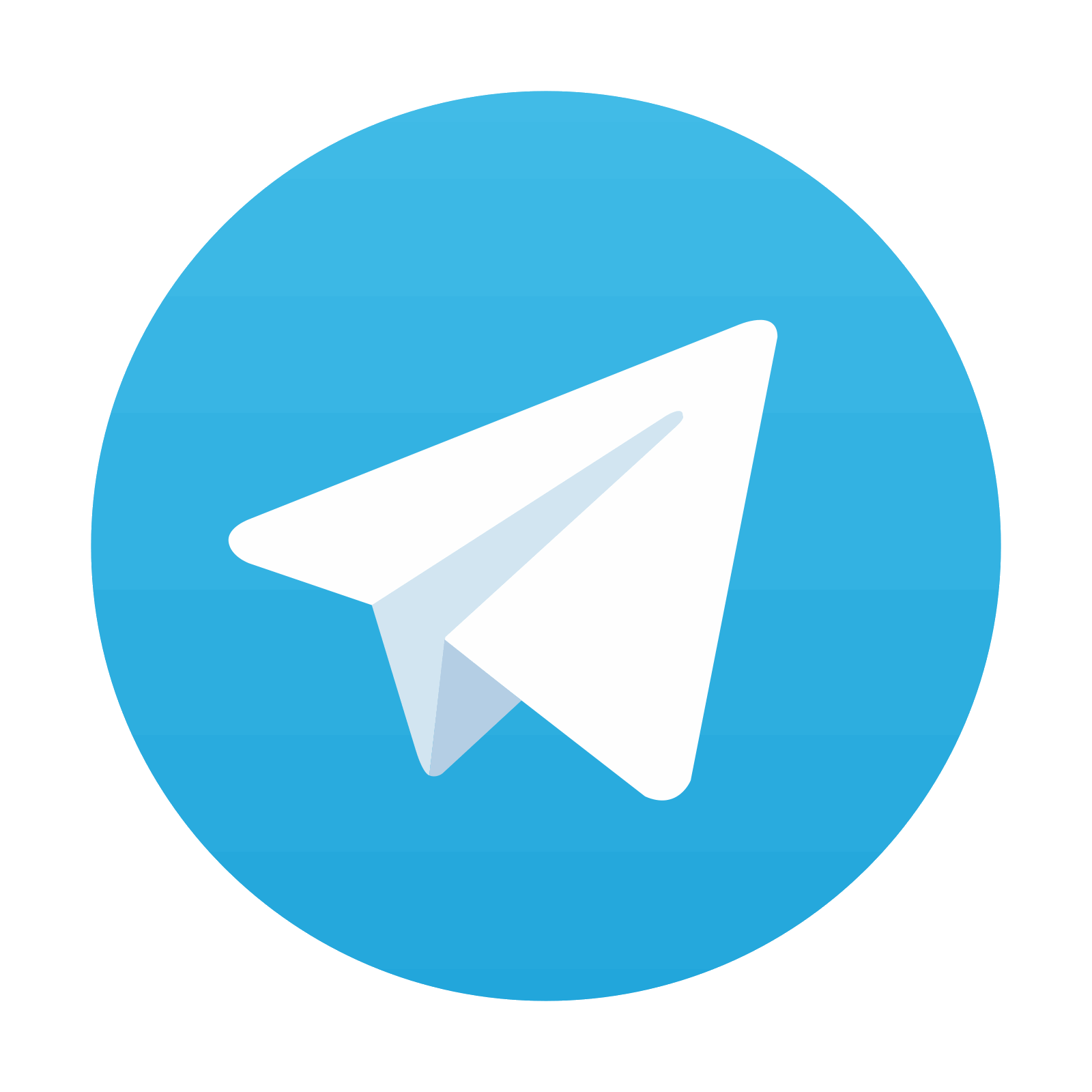
Stay updated, free articles. Join our Telegram channel
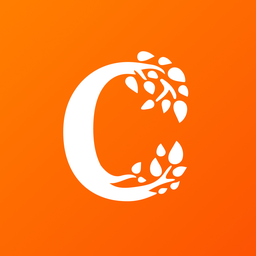
Full access? Get Clinical Tree
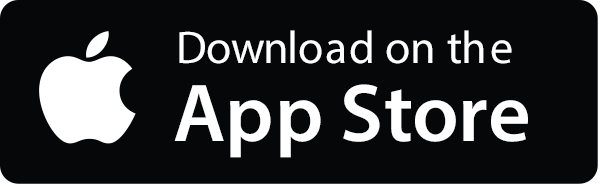
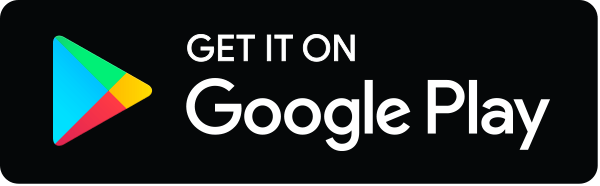