PART 12: Critical Care Medicine
RESPIRATORY CRITICAL CARE |
321 | Approach to the Patient with Critical Illness |
The care of critically ill patients requires a thorough understanding of pathophysiology and centers initially on the resuscitation of patients at the extremes of physiologic deterioration. This resuscitation is often fast-paced and occurs early, without a detailed awareness of the patient’s chronic medical problems. While physiologic stabilization is taking place, intensivists attempt to gather important background medical information to supplement the real-time assessment of the patient’s current physiologic conditions. Numerous tools are available to assist intensivists in the accurate assessment of pathophysiology and management of incipient organ failure, offering a window of opportunity for diagnosing and treating underlying disease(s) in a stabilized patient. Indeed, the use of invasive interventions such as mechanical ventilation and renal replacement therapy is commonplace in the intensive care unit (ICU). An appreciation of the risks and benefits of such aggressive and often invasive interventions is vital to ensure an optimal outcome. Nonetheless, intensivists must recognize when a patient’s chances for recovery are remote or nonexistent and must counsel and comfort dying patients and their significant others. Critical care physicians often must redirect the goals of care from resuscitation and cure to comfort when the resolution of an underlying illness is not possible.
ASSESSMENT OF ILLNESS SEVERITY
In the ICU, illnesses are frequently categorized by degree of severity. Numerous severity-of-illness (SOI) scoring systems have been developed and validated over the past three decades. Although these scoring systems have been validated as tools to assess populations of critically ill patients, their utility in predicting individual patient outcomes is not clear. SOI scoring systems are important for defining populations of critically ill patients. Such systematic scoring allows effective comparison of groups of patients enrolled in clinical trials. In verifying a purported benefit of therapy, investigators must be confident that different groups involved in a clinical trial have similar illness severities. SOI scores are also useful in guiding hospital administrative policies, directing the allocation of resources such as nursing and ancillary care and assisting in assessments of quality of ICU care over time. Scoring system validations are based on the premise that age, chronic medical illnesses, and derangements from normal physiology are associated with increased mortality rates. All existing SOI scoring systems are derived from patients who have already been admitted to the ICU.
SOI scoring systems cannot be used to predict survival in individual patients. No established scoring systems that purport to direct clinicians’ decision-making regarding criteria for admission to an ICU are available, although such models are being developed. Thus the use of SOI scoring systems to direct therapy and clinical decision-making cannot be recommended at present. Instead, these tools should be used as a source of important data to complement clinical bedside decision-making.
The most commonly utilized scoring systems are the APACHE (Acute Physiology and Chronic Health Evaluation) and the SAPS (Simplified Acute Physiology Score) systems.
THE APACHE II SCORING SYSTEM
The APACHE II system is the most commonly used SOI scoring system in North America. Age, type of ICU admission (after elective surgery vs. nonsurgical or after emergency surgery), chronic health problems, and 12 physiologic variables (the worst values for each in the first 24 h after ICU admission) are used to derive a score. The predicted hospital mortality rate is derived from a formula that takes into account the APACHE II score, the need for emergency surgery, and a weighted, disease-specific diagnostic category (Table 321–1). The relationship between APACHE II score and mortality risk is illustrated in Fig. 321-1. Updated versions of the APACHE scoring system (APACHE III and APACHE IV) have been published.
CALCULATION OF ACUTE PHYSIOLOGY AND CHRONIC HEALTH EVALUATION II (APACHE II) SCOREa |
FIGURE 321-1 APACHE II survival curve. Blue, nonoperative; green, postoperative.
THE SAPS SCORING SYSTEM
The SAPS II score, used more frequently in Europe than in the United States, was derived in a manner similar to the APACHE score. This score is not disease specific but rather incorporates three underlying disease variables: AIDS, metastatic cancer, and hematologic malignancy. SAPS 3, which utilizes a 1-h rather than a 24-h window for measuring physiologic derangement scores, was developed in 2005.
SHOCK
See also Chap. 324.
INITIAL EVALUATION
Shock, a common condition necessitating ICU admission or occurring in the course of critical care, is defined by the presence of multisystem end-organ hypoperfusion. Clinical indicators include reduced mean arterial pressure (MAP), tachycardia, tachypnea, cool skin and extremities, acute altered mental status, and oliguria. Hypotension is usually, though not always, present. The end result of multiorgan hypoperfusion is tissue hypoxia, often with accompanying lactic acidosis. Since the MAP is the product of cardiac output and systemic vascular resistance (SVR), reductions in blood pressure can be caused by decreases in cardiac output and/or SVR. Accordingly, once shock is contemplated, the initial evaluation of a hypotensive patient should include an early bedside assessment of the adequacy of cardiac output (Fig. 321-2). Clinical evidence of diminished cardiac output includes a narrow pulse pressure—a marker that correlates with stroke volume—and cool extremities with delayed capillary refill. Signs of increased cardiac output include a widened pulse pressure (particularly with a reduced diastolic pressure), warm extremities with bounding pulses, and rapid capillary refill. If a hypotensive patient has clinical signs of increased cardiac output, it can be inferred that the reduced blood pressure is from decreased SVR.
FIGURE 321-2 Approach to the patient in shock. EGDT, early goal-directed therapy; JVP, jugular venous pulse.
In hypotensive patients with signs of reduced cardiac output, an assessment of intravascular volume status is appropriate. A hypotensive patient with decreased intravascular volume status may have a history suggesting hemorrhage or other volume losses (e.g., vomiting, diarrhea, polyuria). Although evidence of a reduced jugular venous pressure (JVP) is often sought, static measures of right atrial pressure do not predict fluid responsiveness reliably; the change in right atrial pressure as a function of spontaneous respiration is a better predictor of fluid responsiveness (Fig. 321-3). Patients with fluid-responsive (i.e., hypovolemic) shock also may manifest large changes in pulse pressure as a function of respiration during mechanical ventilation (Fig. 321-4). A hypotensive patient with increased intravascular volume and cardiac dysfunction may have S3 and/or S4 gallops on examination, increased JVP, extremity edema, and crackles on lung auscultation. The chest x-ray may show cardiomegaly, widening of the vascular pedicle, Kerley B lines, and pulmonary edema. Chest pain and electrocardiographic changes consistent with ischemia may be noted (Chap. 326).
FIGURE 321-3 Right atrial pressure change during spontaneous respiration in a patient with shock whose cardiac output will increase in response to intravenous fluid administration. The right atrial pressure decreases from 7 mmHg to 4 mmHg. The horizontal bar marks the time of spontaneous inspiration.
FIGURE 321-4 Pulse pressure change during mechanical ventilation in a patient with shock whose cardiac output will increase in response to intravenous fluid administration. The pulse pressure (systolic minus diastolic blood pressure) changes during mechanical ventilation in a patient with septic shock.
In hypotensive patients with clinical evidence of increased cardiac output, a search for causes of decreased SVR is appropriate. The most common cause of high-cardiac-output hypotension is sepsis (Chap. 325). Other causes include liver failure, severe pancreatitis, burns and other trauma that elicit the systemic inflammatory response syndrome (SIRS), anaphylaxis, thyrotoxicosis, and peripheral arteriovenous shunts.
In summary, the most common categories of shock are hypovolemic, cardiogenic, and high-cardiac-output with decreased SVR (high-output hypotension). Certainly more than one category can occur simultaneously (e.g., hypovolemic and septic shock).
The initial assessment of a patient in shock should take only a few minutes. It is important that aggressive resuscitation is instituted on the basis of the initial assessment, particularly since early resuscitation from septic and cardiogenic shock may improve survival (see below). If the initial bedside assessment yields equivocal or confounding data, more objective assessments such as echocardiography and/or invasive vascular monitoring may be useful. The goal of early resuscitation is to reestablish adequate tissue perfusion and thus to prevent or minimize end-organ injury.
MECHANICAL VENTILATORY SUPPORT
(See also Chap. 323) During the initial resuscitation of patients in shock, principles of advanced cardiac life support should be followed. As such patients may be obtunded and unable to protect the airway, an early assessment of the airway is mandatory. Early intubation and mechanical ventilation often are required. Reasons for the institution of endotracheal intubation and mechanical ventilation include acute hypoxemic respiratory failure and ventilatory failure, which frequently accompany shock. Acute hypoxemic respiratory failure may occur in patients with cardiogenic shock and pulmonary edema (Chap. 326) as well as in those who are in septic shock with pneumonia or acute respiratory distress syndrome (ARDS) (Chaps. 322 and 325). Ventilatory failure often occurs as a consequence of an increased load on the respiratory system in the form of acute metabolic (often lactic) acidosis or decreased lung compliance due to pulmonary edema. Inadequate perfusion to respiratory muscles in the setting of shock may be another reason for early intubation and mechanical ventilation. Normally, the respiratory muscles receive a very small percentage of the cardiac output. However, in patients who are in shock with respiratory distress, the percentage of cardiac output dedicated to respiratory muscles may increase by tenfold or more. Lactic acid production from inefficient respiratory muscle activity presents an additional ventilatory load.
Mechanical ventilation may relieve the work of breathing and allow redistribution of a limited cardiac output to other vital organs. Patients demonstrate respiratory distress by an inability to speak full sentences, accessory use of respiratory muscles, paradoxical abdominal muscle activity, extreme tachypnea (>40 breaths/min), and decreasing respiratory rate despite an increasing drive to breathe. When patients with shock are treated with mechanical ventilation, a major goal is for the ventilator to assume all or the majority of the work of breathing, facilitating a state of minimal respiratory muscle work. With the institution of mechanical ventilation for shock, further declines in MAP are frequently seen. The reasons include impeded venous return from positive-pressure ventilation, reduced endogenous catecholamine secretion once the stress associated with respiratory failure abates, and the actions of drugs used to facilitate endotracheal intubation (e.g., propofol, opiates). Accordingly, hypotension should be anticipated during endotracheal intubation. Because many of these patients may be fluid responsive, IV volume administration should be considered. Figure 321-2 summarizes the diagnosis and treatment of different types of shock For further discussion of individual forms of shock, see Chaps. 324, 325, and 326.
RESPIRATORY FAILURE
Respiratory failure is one of the most common reasons for ICU admission. In some ICUs, ≥75% of patients require mechanical ventilation during their stay. Respiratory failure can be categorized mechanistically on the basis of pathophysiologic derangements in respiratory function.
TYPE I: ACUTE HYPOXEMIC RESPIRATORY FAILURE
This type of respiratory failure occurs with alveolar flooding and subsequent intrapulmonary shunt physiology. Alveolar flooding may be a consequence of pulmonary edema, pneumonia, or alveolar hemorrhage. Pulmonary edema can be further categorized as occurring due to elevated pulmonary microvascular pressures, as seen in heart failure and intravascular volume overload or ARDS (“low-pressure pulmonary edema,” Chap. 322). This syndrome is defined by acute onset (≤1 week) of bilateral opacities on chest imaging that are not fully explained by cardiac failure or fluid overload and of shunt physiology requiring positive end-expiratory pressure (PEEP). Type I respiratory failure occurs in clinical settings such as sepsis, gastric aspiration, pneumonia, near-drowning, multiple blood transfusions, and pancreatitis. The mortality rate among patients with ARDS was traditionally very high (50–70%), although changes in patient care have led to mortality rates closer to 30% (see below).
For many years, physicians have suspected that mechanical ventilation of patients with ARDS may propagate lung injury. Cyclical collapse and reopening of alveoli may be partly responsible for this adverse effect. As seen in Fig. 321-5, the pressure-volume relationship of the lung in ARDS is not linear. Alveoli may collapse at very low lung volumes. Animal studies have suggested that stretching and overdistention of injured alveoli during mechanical ventilation can further injure the lung. Concern over this alveolar overdistention, termed ventilator-induced “volutrauma,” led to a multicenter, randomized, prospective trial comparing traditional ventilator strategies for ARDS (large tidal volume: 12 mL/kg of ideal body weight) with a low tidal volume (6 mL/kg of ideal body weight). This study showed a dramatic reduction in mortality rate in the low-tidal-volume group from that in the high-tidal-volume group (31% versus 39.8%). In addition, a “fluid-conservative” management strategy (maintaining a low central venous pressure [CVP] or pulmonary capillary wedge pressure [PCWP]) is associated with fewer days of mechanical ventilation than a “fluid-liberal” strategy (maintaining a relatively high CVP or PCWP) in ARDS.
FIGURE 321-5 Pressure-volume relationship in the lungs of a patient with acute respiratory distress syndrome (ARDS). At the lower inflection point, collapsed alveoli begin to open and lung compliance changes. At the upper deflection point, alveoli become overdistended. The shape and size of alveoli are illustrated at the top of the figure.
TYPE II RESPIRATORY FAILURE
This type of respiratory failure is a consequence of alveolar hypoventilation and results from the inability to eliminate carbon dioxide effectively. Mechanisms are categorized by impaired central nervous system (CNS) drive to breathe, impaired strength with failure of neuromuscular function in the respiratory system, and increased load(s) on the respiratory system. Reasons for diminished CNS drive to breathe include drug overdose, brainstem injury, sleep-disordered breathing, and severe hypothyroidism. Reduced strength can be due to impaired neuromuscular transmission (e.g., myasthenia gravis, Guillain-Barré syndrome, amyotrophic lateral sclerosis) or respiratory muscle weakness (e.g., myopathy, electrolyte derangements, fatigue).
The overall load on the respiratory system can be subclassified into resistive loads (e.g., bronchospasm), loads due to reduced lung compliance (e.g., alveolar edema, atelectasis, intrinsic positive end-expiratory pressure [auto-PEEP]—see below), loads due to reduced chest wall compliance (e.g., pneumothorax, pleural effusion, abdominal distention), and loads due to increased minute ventilation requirements (e.g., pulmonary embolus with increased dead-space fraction, sepsis).
The mainstays of therapy for type II respiratory failure are directed at reversing the underlying cause(s) of ventilatory failure. Noninvasive positive-pressure ventilation with a tight-fitting facial or nasal mask, with avoidance of endotracheal intubation, often stabilizes these patients. This approach has been shown to be beneficial in treating patients with exacerbations of chronic obstructive pulmonary disease; it has been tested less extensively in other kinds of respiratory failure but may be attempted nonetheless in the absence of contraindications (hemodynamic instability, inability to protect the airway, respiratory arrest).
TYPE III RESPIRATORY FAILURE
This form of respiratory failure results from lung atelectasis. Because atelectasis occurs so commonly in the perioperative period, this form is also called perioperative respiratory failure. After general anesthesia, decreases in functional residual capacity lead to collapse of dependent lung units. Such atelectasis can be treated by frequent changes in position, chest physiotherapy, upright positioning, and control of incisional and/or abdominal pain. Noninvasive positive-pressure ventilation may also be used to reverse regional atelectasis.
TYPE IV RESPIRATORY FAILURE
This form results from hypoperfusion of respiratory muscles in patients in shock. Normally, respiratory muscles consume <5% of total cardiac output and oxygen delivery. Patients in shock often experience respiratory distress due to pulmonary edema (e.g., in cardiogenic shock), lactic acidosis, and anemia. In this setting, up to 40% of cardiac output may be distributed to the respiratory muscles. Intubation and mechanical ventilation can allow redistribution of the cardiac output away from the respiratory muscles and back to vital organs while the shock is treated.
CARE OF THE MECHANICALLY VENTILATED PATIENT
(See also Chap. 323) Whereas a thorough understanding of the pathophysiology of respiratory failure is essential for optimal patient care, recognition of a patient’s readiness to be liberated from mechanical ventilation is likewise important. Several studies have shown that daily spontaneous breathing trials can identify patients who are ready for extubation. Accordingly, all intubated, mechanically ventilated patients should undergo daily screening of respiratory function. If oxygenation is stable (i.e., PaO2/FIO2 [partial pressure of oxygen/fraction of inspired oxygen] >200 and PEEP ≤5 cmH2O), cough and airway reflexes are intact, and no vasopressor agents or sedatives are being administered, the patient has passed the screening test and should undergo a spontaneous breathing trial. This trial consists of a period of breathing through the endotracheal tube without ventilator support (both continuous positive airway pressure [CPAP] of 5 cmH2O and an open T-piece breathing system can be used) for 30–120 min. The spontaneous breathing trial is declared a failure and stopped if any of the following occur: (1) respiratory rate >35/min for >5 min, (2) O2 saturation <90%, (3) heart rate >140/min or a 20% increase or decrease from baseline, (4) systolic blood pressure <90 mmHg or >180 mmHg, or (5) increased anxiety or diaphoresis. If, at the end of the spontaneous breathing trial, none of the above events has occurred and the ratio of the respiratory rate and tidal volume in liters (f/VT) is <105, the patient can be extubated. Such protocol-driven approaches to patient care can have an important impact on the duration of mechanical ventilation and ICU stay. In spite of such a careful approach to liberation from mechanical ventilation, up to 10% of patients develop respiratory distress after extubation and may require resumption of mechanical ventilation. Many of these patients will require reintubation. The use of noninvasive ventilation in patients in whom extubation fails may be associated with worse outcomes than are obtained with immediate reintubation.
Mechanically ventilated patients frequently require sedatives and analgesics. Opiates are the mainstay of therapy for pain control in mechanically ventilated patients. After adequate pain control has been ensured, additional indications for sedation include anxiolysis; treatment of subjective dyspnea; psychosis; facilitation of nursing care; reduction of autonomic hyperactivity, which may precipitate myocardial ischemia; and reduction of total O2 consumption (VO2).
Neuromuscular blocking agents are occasionally needed to facilitate mechanical ventilation in patients with profound ventilator dyssynchrony despite optimal sedation, particularly in the setting of severe ARDS. Use of these agents may result in prolonged weakness—a myopathy known as the postparalytic syndrome. For this reason, neuromuscular blocking agents typically are used as a last resort when aggressive sedation fails to achieve patient-ventilator synchrony. Because neuromuscular blocking agents result in pharmacologic paralysis without altering mental status, sedative-induced amnesia is mandatory when these agents are administered.
Amnesia can be achieved reliably with benzodiazepines such as lorazepam and midazolam as well as the the IV anesthetic agent propofol. Outside the setting of pharmacologic paralysis, few data support the idea that amnesia is mandatory in all patients who require intubation and mechanical ventilation. Since many of these critically patients have impaired hepatic and renal function, sedatives and opiates may accumulate when given for prolonged periods. A nursing protocol–driven approach to sedation of mechanically ventilated patients or daily interruption of sedative infusions paired with daily spontaneous breathing trials has been shown to prevent excessive drug accumulation and shorten the duration of both mechanical ventilation and ICU stay.
MULTIORGAN SYSTEM FAILURE
Multiorgan system failure, which is commonly associated with critical illness, is defined by the simultaneous presence of physiologic dysfunction and/or failure of two or more organs. Typically, this syndrome occurs in the setting of severe sepsis, shock of any kind, severe inflammatory conditions such as pancreatitis, and trauma. The fact that multiorgan system failure occurs commonly in the ICU is a testament to our current ability to stabilize and support single-organ failure. The ability to support single-organ failure aggressively (e.g., by mechanical ventilation or by renal replacement therapy) has reduced rates of early mortality in critical illness. As a result, it is uncommon for critically ill patients to die in the initial stages of resuscitation. Instead, many patients succumb to critical illness later in the ICU stay, after the initial presenting problem has been stabilized.
Although there is debate regarding specific definitions of organ failure, several general principles governing the syndrome of multiorgan system failure apply. First, organ failure, no matter how it is defined, must persist beyond 24 h. Second, mortality risk increases with the accrual of failing organs. Third, the prognosis worsens with increased duration of organ failure. These observations remain true across various critical care settings (e.g., medical versus surgical). SIRS is a common basis for multiorgan system failure. Although infection is a common cause of SIRS, “sterile” triggers such as pancreatitis, trauma, and burns often are invoked to explain multiorgan system failure.
MONITORING IN THE ICU
Because respiratory failure and circulatory failure are common in critically ill patients, monitoring of the respiratory and cardiovascular systems is undertaken frequently. Evaluation of respiratory gas exchange is routine in critical illness. The “gold standard” remains arterial blood-gas analysis, in which pH, PaO2, partial pressure of carbon dioxide (PCO2), and O2 saturation are measured directly. With arterial blood-gas analysis, the two main functions of the lung—oxygenation of arterial blood and elimination of CO2—can be assessed directly. In fact, the blood pH, which has a profound effect on the drive to breathe, can be assessed only by such sampling. Although sampling of arterial blood is generally safe, it may be painful and cannot provide continuous information. In light of these limitations, noninvasive monitoring of respiratory function is often employed.
PULSE OXIMETRY
The most commonly utilized noninvasive technique for monitoring respiratory function, pulse oximetry takes advantage of differences in the absorptive properties of oxygenated and deoxygenated hemoglobin. At wavelengths of 660 nm, oxyhemoglobin reflects light more effectively than does deoxyhemoglobin, whereas the reverse is true in the infrared spectrum (940 nm). A pulse oximeter passes both wavelengths of light through a perfused digit such as a finger, and the relative intensity of light transmission at these two wavelengths is recorded. From this information, the relative percentage of oxyhemoglobin is derived. Since arterial pulsations produce phasic changes in the intensity of transmitted light, the pulse oximeter is designed to detect only light of alternating intensity. This feature allows distinction of arterial and venous blood O2 saturations.
RESPIRATORY SYSTEM MECHANICS
Respiratory system mechanics can be measured in patients during mechanical ventilation (Chap. 323). When volume-controlled modes of mechanical ventilation are used, accompanying airway pressures can easily be measured as long as the patient is passive. The peak airway pressure is determined by two variables: airway resistance and respiratory system compliance. At the end of inspiration, inspiratory flow can be stopped transiently. This end-inspiratory pause (plateau pressure) is a static measurement, affected only by respiratory system compliance and not by airway resistance. Therefore, during volume-controlled ventilation, the difference between the peak (airway resistance + respiratory system compliance) and plateau (respiratory system compliance only) airway pressures provides a quantitative assessment of airway resistance. Accordingly, during volume-controlled ventilation, patients with increases in airway resistance typically have increased peak airway pressures as well as abnormally high gradients between peak and plateau airway pressures (typically >15 cmH2O) at an inspiratory flow rate of 1 L/sec. The compliance of the respiratory system is defined by the change in pressure of the respiratory system per unit change in volume.
The respiratory system can be divided into two components: the lungs and the chest wall. Normally, respiratory system compliance is ~100 mL/cmH2O. Pathophysiologic processes such as pleural effusions, pneumothorax, and increased abdominal girth all reduce chest wall compliance. Lung compliance may be reduced by pneumonia, pulmonary edema, interstitial lung disease, or auto-PEEP. Accordingly, patients with abnormalities in compliance of the respiratory system (lungs and/or chest wall) typically have elevated peak and plateau airway pressures but a normal gradient between these two pressures. Auto-PEEP occurs when there is insufficient time for emptying of alveoli before the next inspiratory cycle. Since the alveoli have not decompressed completely, alveolar pressure remains positive at the end of exhalation (functional residual capacity). This phenomenon results most commonly from critical narrowing of distal airways in disease processes such as asthma and COPD. Auto-PEEP with resulting alveolar overdistention may result in diminished lung compliance, reflected by abnormally increased plateau airway pressures. Modern mechanical ventilators allow breath-to-breath display of pressure and flow, permitting detection of problems such as patient-ventilator dyssynchrony, airflow obstruction, and auto-PEEP (Fig. 321–6).
FIGURE 321-6 Increased airway resistance with auto-PEEP. The top waveform (airway pressure vs. time) shows a large difference between the peak airway pressure (80 cmH2O) and the plateau airway pressure (20 cmH2O). The bottom waveform (flow vs. time) demonstrates airflow throughout expiration (reflected by the flow tracing on the negative portion of the abscissa) that persists up to the next inspiratory effort.
CIRCULATORY STATUS
Oxygen delivery (QO2) is a function of cardiac output and the content of O2 in the arterial blood (CaO2). The CaO2 is determined by the hemoglobin concentration, the arterial hemoglobin saturation, and dissolved O2 not bound to hemoglobin. For normal adults:
QO2 = 50 dL/min × (1.39 × 15 g/dL [hemoglobin concentration] × 1.0 [hemoglobin % saturation] + 0.0031 × 100 [PaO2])
= 50 dL/min (cardiac output) × 21.6 mL O2 per dL blood (CaO2)
= 1058 mL O2 per min
It is apparent that nearly all of the O2 delivered to tissues is bound to hemoglobin and that the dissolved O2 (PaO2) contributes very little to O2 content in arterial blood or to O2 delivery. Normally, the content of O2 in mixed venous blood (CO2) is 15.76 mL/dL since the mixed venous blood is 75% saturated. Therefore, the normal tissue extraction ratio for O2 is CaO2 – C
O2/CaO2 ([21.16–15.76]/21.16) or ~25%. A pulmonary artery catheter allows measurements of O2 delivery and the O2 extraction ratio.
Information on the mixed venous O2 saturation allows assessment of global tissue perfusion. A reduced mixed venous O2 saturation may be caused by inadequate cardiac output, reduced hemoglobin concentration, and/or reduced arterial O2 saturation. An abnormally high VO2 may also lead to a reduced mixed venous O2 saturation if O2 delivery is not concomitantly increased. Abnormally increased VO2 in peripheral tissues may be caused by problems such as fever, agitation, shivering, and thyrotoxicosis.
The pulmonary artery catheter originally was designed as a tool to guide therapy for acute myocardial infarction but has been used in the ICU for evaluation and treatment of a variety of other conditions, such as ARDS, septic shock, congestive heart failure, and acute renal failure. This device has never been validated as a tool associated with reduction in morbidity and mortality rates. Indeed, despite numerous prospective studies, mortality or morbidity rate benefits associated with use of the pulmonary artery catheter have never been reported in any setting. Accordingly, it appears that routine pulmonary artery catheterization is not indicated as a means of monitoring and characterizing circulatory status in most critically ill patients.
Static measurements of circulatory parameters (e.g., CVP, PCWP) do not provide reliable information on the circulatory status of critically ill patients. In contrast, dynamic assessments measuring the impact of breathing on the circulation are more reliable predictors of responsiveness to IV fluid administration. A decrease in CVP of >1 mmHg during inspiration in a spontaneously breathing patient may predict an increase in cardiac output after IV fluid administration. Similarly, a changing pulse pressure during mechanical ventilation has been shown to predict an increase in cardiac output after IV fluid administration in patients with septic shock.
PREVENTION OF COMPLICATIONS OF CRITICAL ILLNESS
SEPSIS IN THE CRITICAL CARE UNIT
(See also Chap. 325) Sepsis, defined as the presence of SIRS in the setting of known or suspected infection, is a significant problem in the care of critically ill patients, who often progress to severe sepsis with the failure of one or more organs. Sepsis is the leading cause of death in noncoronary ICUs in the United States, with case rates expected to increase as the population ages and a higher percentage of people are vulnerable to infection.
NOSOCOMIAL INFECTIONS IN THE ICU
Many therapeutic interventions in the ICU are invasive and predispose patients to infectious complications. These interventions include endotracheal intubation, indwelling vascular catheters, transurethral bladder catheters, and other catheters placed into sterile body cavities (e.g., tube thoracostomy, percutaneous intraabdominal drainage catheterization). The longer such devices remain in place, the more prone to these infections patients become. For example, ventilator-associated pneumonia correlates strongly with the duration of intubation and mechanical ventilation. Therefore, an important aspect of preventive care is the timely removal of invasive devices as soon as they are no longer needed. Moreover, multidrug-resistant organisms are commonplace in the ICU.
Infection control is critical in the ICU. Care bundles, which include measures such as frequent hand washing, are effective but underutilized strategies. Other components of care bundles, such as protective isolation of patients colonized or infected by drug-resistant organisms, are also commonly used. Silver-coated endotracheal tubes reportedly reduce the incidence of ventilator-associated pneumonia. Studies evaluating multifaceted, evidence-based strategies to decrease catheter-related bloodstream infections have shown improved outcomes with strict adherence to measures such as hand washing, full-barrier precautions during catheter insertion, chlorhexidine skin preparation, avoidance of the femoral site, and timely catheter removal.
DEEP VENOUS THROMBOSIS (DVT)
(See also Chap. 300) All ICU patients are at high risk for this complication because of their predilection for immobility. Therefore, all should receive some form of prophylaxis against DVT. The most commonly employed forms of prophylaxis are subcutaneous low-dose heparin injections and sequential compression devices for the lower extremities. Observational studies report an alarming incidence of DVTs despite the use of these standard prophylactic regimens. Furthermore, heparin prophylaxis may result in heparin-induced thrombocytopenia, another nosocomial complication in critically ill patients.
Low-molecular-weight heparins such as enoxaparin are more effective than unfractionated heparin for DVT prophylaxis in high-risk patients (e.g., those undergoing orthopedic surgery) and are associated with a lower incidence of heparin-induced thrombocytopenia. Fondaparinux, a selective factor Xa inhibitor, is even more effective than enoxaparin in high-risk orthopedic patients.
STRESS ULCERS
Prophylaxis against stress ulcers is frequently administered in most ICUs; typically, histamine-2 antagonists or proton pump inhibitors are given. Available data suggest that high-risk patients, such as those with coagulopathy, shock, or respiratory failure requiring mechanical ventilation, benefit from such prophylactic treatment.
NUTRITION AND GLYCEMIC CONTROL
These are important issues that may be associated with respiratory failure, impaired wound healing, and dysfunctional immune response in critically ill patients. Early enteral feeding is reasonable, though no data are available to suggest that this treatment improves patient outcome per se. Certainly, enteral feeding, if possible, is preferred over parenteral nutrition, which is associated with numerous complications, including hyperglycemia, fatty liver, cholestasis, and sepsis. When parenteral feeding is necessary to supplement enteral nutrition, delaying this intervention until day 8 in the ICU results in better recovery and fewer ICU-related complications. Tight glucose control is an area of controversy in critical care. Although one study showed a significant mortality benefit when glucose levels were aggressively normalized in a large group of surgical ICU patients, more recent data for a large population of both medical and surgical ICU patients suggested that tight glucose control resulted in increased rates of mortality.
ICU-ACQUIRED WEAKNESS
ICU-acquired weakness occurs frequently in patients who survive critical illness, particularly those with SIRS and/or sepsis. Both neuropathies and myopathies have been described, most commonly after ~1 week in the ICU. The mechanisms behind ICU-acquired weakness syndromes are poorly understood. Intensive insulin therapy may reduce polyneuropathy in critical illness. Very early physical and occupational therapy in mechanically ventilated patients reportedly results in significant improvements in functional independence at hospital discharge as well as in reduced durations of mechanical ventilation and delirium.
ANEMIA
Studies have shown that most ICU patients are anemic as a result of chronic inflammation. Phlebotomy also contributes to ICU anemia. A large multicenter study involving patients in many different ICU settings challenged the conventional notion that a hemoglobin level of 100 g/L (10 g/dL) is needed in critically ill patients, with similar outcomes noted in those whose transfusion trigger was 7 g/dL. Red blood cell transfusion is associated with impairment of immune function and increased risk of infections as well as of ARDS and volume overload, all of which may explain the findings in this study. Recently, a conservative transfusion strategy enhanced survival among patients with active upper gastrointestinal hemorrhage.
ACUTE RENAL FAILURE
(See also Chap. 334) Acute renal failure occurs in a significant percentage of critically ill patients. The most common underlying etiology is acute tubular necrosis, usually precipitated by hypoperfusion and/or nephrotoxic agents. Currently, no pharmacologic agents are available for prevention of renal injury in critical illness. Studies have shown convincingly that low-dose dopamine is not effective in protecting the kidneys from acute injury.
NEUROLOGIC DYSFUNCTION IN CRITICALLY ILL PATIENTS
DELIRIUM
(See also Chaps. 34 and 328) This state is defined by (1) an acute onset of changes or fluctuations in mental status, (2) inattention, (3) disorganized thinking, and (4) an altered level of consciousness (i.e., a state other than alertness). Delirium is reported to occur in a wide range of mechanically ventilated ICU patients and can be detected by the Confusion Assessment Method (CAM)-ICU or the Intensive Care Delirium Screening Checklist. These tools are used to ask patients to answer simple questions and perform simple tasks and can be used readily at the bedside. The differential diagnosis of delirium in ICU patients is broad and includes infectious etiologies (including sepsis), medications (particularly sedatives and analgesics), drug withdrawal, metabolic/electrolyte derangements, intracranial pathology (e.g., stroke, intracranial hemorrhage), seizures, hypoxia, hypertensive crisis, shock, and vitamin deficiencies (particularly thiamine). Patients with ICU delirium have increases in length of hospital stay, time on mechanical ventilation, cognitive impairment at hospital discharge, and 6-month mortality rate. Interventions to reduce ICU delirium are limited. The sedative dexmedetomidine has been less strongly associated with ICU delirium than midazolam. In addition, as mentioned above, very early physical and occupational therapy in mechanically ventilated patients has been demonstrated to reduce delirium.
ANOXIC CEREBRAL INJURY
(See also Chap. 330) This condition is common after cardiac arrest and often results in severe and permanent brain injury in survivors. Active cooling of patients after cardiac arrest has been shown to improve neurologic outcomes. Therefore, patients who present to the ICU after circulatory arrest from ventricular fibrillation or pulseless ventricular tachycardia should be actively cooled to achieve a core body temperature of 32–34°C.
STROKE
(See also Chap. 446) Stroke is a common cause of neurologic critical illness. Hypertension must be managed carefully, since abrupt reductions in blood pressure may be associated with further brain ischemia and injury. Acute ischemic stroke treated with tissue plasminogen activator (tPA) has an improved neurologic outcome when treatment is given within 3 h of onset of symptoms. The mortality rate is not reduced when tPA is compared with placebo, despite the improved neurologic outcome. The risk of cerebral hemorrhage is significantly higher in patients given tPA. No benefit is seen when tPA therapy is given beyond 3 h after symptom onset. Heparin has not been convincingly shown to improve outcomes in patients with acute ischemic stroke. Decompressive craniectomy is a surgical procedure that relieves increased intracranial pressure in the setting of space-occupying brain lesions or brain swelling from stroke; available evidence suggests that this procedure may improve survival among select patients (≤55 years or age), albeit at a cost of increased disability for some.
SUBARACHNOID HEMORRHAGE
(See also Chap. 446) Subarachnoid hemorrhage may occur secondary to aneurysm rupture and is often complicated by cerebral vasospasm, re-bleeding, and hydrocephalus. Vasospasm can be detected by either transcranial Doppler assessment or cerebral angiography; it is typically treated with the calcium channel blocker nimodipine, aggressive IV fluid administration, and therapy aimed at increasing blood pressure, typically with vasoactive drugs such as phenylephrine. The IV fluids and vasoactive drugs (hypertensive hypervolemic therapy) are used to overcome the cerebral vasospasm. Early surgical clipping or endovascular coiling of aneurysms is advocated to prevent complications related to re-bleeding. Hydrocephalus, typically heralded by a decreased level of consciousness, may require ventriculostomy drainage.
STATUS EPILEPTICUS
(See also Chap. 445) Recurrent or relentless seizure activity is a medical emergency. Cessation of seizure activity is required to prevent irreversible neurologic injury. Lorazepam is the most effective benzodiazepine for treating status epilepticus and is the treatment of choice for controlling seizures acutely. Phenytoin or fosphenytoin should be given concomitantly since lorazepam has a short half-life. Other drugs, such as gabapentin, carbamazepine, and phenobarbital, should be reserved for patients with contraindications to phenytoin (e.g., allergy or pregnancy) or ongoing seizures despite phenytoin.
BRAIN DEATH
(See also Chap. 330) Although deaths of critically ill patients usually are attributable to irreversible cessation of circulatory and respiratory function, a diagnosis of death also may be established by irreversible cessation of all functions of the entire brain, including the brainstem, even if circulatory and respiratory functions remain intact on artificial life support. Such a diagnosis requires demonstration of the absence of cerebral function (no response to any external stimulus) and brainstem functions (e.g., unreactive pupils, lack of ocular movement in response to head turning or ice-water irrigation of ear canals, positive apnea test [no drive to breathe]). Absence of brain function must have an established cause and be permanent without possibility of recovery; a sedative effect, hypothermia, hypoxemia, neuromuscular paralysis, and severe hypotension must be ruled out. If there is uncertainty about the cause of coma, studies of cerebral blood flow and electroencephalography should be performed.
WITHHOLDING OR WITHDRAWING CARE
(See also Chap. 10) Withholding or withdrawal of care occurs commonly in the ICU setting. The Task Force on Ethics of the Society of Critical Care Medicine reported that it is ethically sound to withhold or withdraw care if a patient or the patient’s surrogate makes such a request or if the physician judges that the goals of therapy are not achievable. Since all medical treatments are justified by their expected benefits, the loss of such an expectation justifies the act of withdrawing or withholding such treatment; these two actions are judged to be fundamentally similar. An underlying stipulation derived from this report is that an informed patient should have his or her wishes respected with regard to life-sustaining therapy. Implicit in this stipulation is the need to ensure that patients are thoroughly and accurately informed regarding the plausibility and expected results of various therapies.
The act of informing patients and/or surrogate decision-makers is the responsibility of the physician and other health care providers. If a patient or surrogate desires therapy deemed futile by the treating physician, the physician is not obligated ethically to provide such treatment. Rather, arrangements may be made to transfer the patient’s care to another care provider. Whether the decision to withdraw life support should be initiated by the physician or left to surrogate decision-makers alone is not clear. One study reported that slightly more than half of surrogate decision-makers preferred to receive such a recommendation, whereas the rest did not. Critical care providers should meet regularly with patients and/or surrogates to discuss prognosis when the withholding or withdrawal of care is being considered. After a consensus among caregivers has been reached, this information should be relayed to the patient and/or surrogate decision-maker. If a decision to withhold or withdraw life-sustaining care for a patient has been made, aggressive attention to analgesia and anxiolysis is needed.
322 | Acute Respiratory Distress Syndrome |
Acute respiratory distress syndrome (ARDS) is a clinical syndrome of severe dyspnea of rapid onset, hypoxemia, and diffuse pulmonary infiltrates leading to respiratory failure. ARDS is caused by diffuse lung injury from many underlying medical and surgical disorders. The lung injury may be direct, as occurs in toxic inhalation, or indirect, as occurs in sepsis (Table 322-1). The clinical features of ARDS are listed in Table 322-2. By expert consensus, ARDS is defined by three categories based on the degrees of hypoxemia (Table 322-2). These stages of mild, moderate, and severe ARDS are associated with mortality risk and with the duration of mechanical ventilation in survivors.
CLINICAL DISORDERS COMMONLY ASSOCIATED WITH ARDS |
DIAGNOSTIC CRITERIA FOR ARDS |
The annual incidence of ARDS is estimated to be as high as 60 cases/100,000 population. Approximately 10% of all intensive care unit (ICU) admissions involve patients with acute respiratory failure; ~20% of these patients meet the criteria for ARDS.
ETIOLOGY
While many medical and surgical illnesses have been associated with the development of ARDS, most cases (>80%) are caused by a relatively small number of clinical disorders: severe sepsis syndrome and/or bacterial pneumonia (~40–50%), trauma, multiple transfusions, aspiration of gastric contents, and drug overdose. Among patients with trauma, the most frequently reported surgical conditions in ARDS are pulmonary contusion, multiple bone fractures, and chest wall trauma/flail chest, whereas head trauma, near-drowning, toxic inhalation, and burns are rare causes. The risks of developing ARDS are increased in patients with more than one predisposing medical or surgical condition.
Several other clinical variables have been associated with the development of ARDS. These include older age, chronic alcohol abuse, metabolic acidosis, and severity of critical illness. Trauma patients with an Acute Physiology and Chronic Health Evaluation (APACHE) II score ≥16 (Chap. 321) have a 2.5-fold increased risk of developing ARDS, and those with a score >20 have an incidence of ARDS that is more than threefold greater than the incidence among those with APACHE II scores ≤9.
CLINICAL COURSE AND PATHOPHYSIOLOGY
The natural history of ARDS is marked by three phases—exudative, proliferative, and fibrotic—that each have characteristic clinical and pathologic features (Fig. 322-1).
FIGURE 322-1 Diagram illustrating the time course for the development and resolution of ARDS. The exudative phase is notable for early alveolar edema and neutrophil-rich leukocytic infiltration of the lungs, with subsequent formation of hyaline membranes from diffuse alveolar damage. Within 7 days, a proliferative phase ensues with prominent interstitial inflammation and early fibrotic changes. Approximately 3 weeks after the initial pulmonary injury, most patients recover. However, some patients enter the fibrotic phase, with substantial fibrosis and bullae formation.
Exudative Phase In this phase (Fig. 322-2), alveolar capillary endothelial cells and type I pneumocytes (alve\olar epithelial cells) are injured, with consequent loss of the normally tight alveolar barrier to fluid and macromolecules. Edema fluid that is rich in protein accumulates in the interstitial and alveolar spaces. Significant concentrations of cytokines (e.g., interleukin 1, interleukin 8, and tumor necrosis factor α) and lipid mediators (e.g., leukotriene B4) are present in the lung in this acute phase. In response to proinflammatory mediators, leukocytes (especially neutrophils) traffic into the pulmonary interstitium and alveoli. In addition, condensed plasma proteins aggregate in the air spaces with cellular debris and dysfunctional pulmonary surfactant to form hyaline membrane whorls. Pulmonary vascular injury also occurs early in ARDS, with vascular obliteration by microthrombi and fibrocellular proliferation (Fig. 322-3).
FIGURE 322-2 A representative anteroposterior chest x-ray in the exudative phase of ARDS shows diffuse interstitial and alveolar infiltrates that can be difficult to distinguish from left ventricular failure.
FIGURE 322-3 The normal alveolus (left) and the injured alveolus in the acute phase of acute lung injury and the acute respiratory distress syndrome (right). In the acute phase of the syndrome (right), there is sloughing of both the bronchial and alveolar epithelial cells, with the formation of protein-rich hyaline membranes on the denuded basement membrane. Neutrophils are shown adhering to the injured capillary endothelium and transmigrating through the interstitium into the air space, which is filled with protein-rich edema fluid. In the air space, an alveolar macrophage is secreting cytokines—i.e., interleukins 1, 6, 8, and 10 (IL-1, -6, -8, and -10) and tumor necrosis factor α (TNF-α)—that act locally to stimulate chemotaxis and activate neutrophils. Macrophages also secrete other cytokines, including IL-1, -6, and -10. IL-1 can also stimulate the production of extracellular matrix by fibroblasts. Neutrophils can release oxidants, proteases, leukotrienes, and other proinflammatory molecules, such as platelet-activating factor (PAF). A number of antiinflammatory mediators are also present in the alveolar milieu, including the IL-1-receptor antagonist, soluble TNF-α receptor, autoantibodies to IL-8, and cytokines such as IL-10 and IL-11 (not shown). The influx of protein-rich edema fluid into the alveolus has led to the inactivation of surfactant. MIF, macrophage inhibitory factor. (From LB Ware, MA Matthay: N Engl J Med 342:1334, 2000, with permission.)
Alveolar edema predominantly involves dependent portions of the lung, with diminished aeration and atelectasis. Collapse of large sections of dependent lung markedly decreases lung compliance. Consequently, intrapulmonary shunting and hypoxemia develop and the work of breathing increases, leading to dyspnea. The pathophysiologic alterations in alveolar spaces are exacerbated by microvascular occlusion that results in reductions in pulmonary arterial blood flow to ventilated portions of the lung (and thus in increased dead space) and in pulmonary hypertension. Thus, in addition to severe hypoxemia, hypercapnia secondary to an increase in pulmonary dead space is prominent in early ARDS.
The exudative phase encompasses the first 7 days of illness after exposure to a precipitating ARDS risk factor, with the patient experiencing the onset of respiratory symptoms. Although usually presenting within 12–36 h after the initial insult, symptoms can be delayed by 5–7 days. Dyspnea develops, with a sensation of rapid shallow breathing and an inability to get enough air. Tachypnea and increased work of breathing result frequently in respiratory fatigue and ultimately in respiratory failure. Laboratory values are generally nonspecific and are primarily indicative of underlying clinical disorders. The chest radiograph usually reveals alveolar and interstitial opacities involving at least three-quarters of the lung fields (Fig. 322-2). While characteristic for ARDS, these radiographic findings are not specific and can be indistinguishable from cardiogenic pulmonary edema (Chap. 326). Unlike the latter, however, the chest x-ray in ARDS rarely shows cardiomegaly, pleural effusions, or pulmonary vascular redistribution. Chest CT in ARDS reveals extensive heterogeneity of lung involvement (Fig. 322-4).
FIGURE 322-4 A representative CT scan of the chest during the exudative phase of ARDS, in which dependent alveolar edema and atelectasis predominate.
Because the early features of ARDS are nonspecific, alternative diagnoses must be considered. In the differential diagnosis of ARDS, the most common disorders are cardiogenic pulmonary edema, diffuse pneumonia, and alveolar hemorrhage. Less common diagnoses to consider include acute interstitial lung diseases (e.g., acute interstitial pneumonitis; Chap. 315), acute immunologic injury (e.g., hypersensitivity pneumonitis; Chap. 310), toxin injury (e.g., radiation pneumonitis; Chap. 263), and neurogenic pulmonary edema (Chap. 47e).
Proliferative Phase This phase of ARDS usually lasts from day 7 to day 21. Most patients recover rapidly and are liberated from mechanical ventilation during this phase. Despite this improvement, many patients still experience dyspnea, tachypnea, and hypoxemia. Some patients develop progressive lung injury and early changes of pulmonary fibrosis during the proliferative phase. Histologically, the first signs of resolution are often evident in this phase, with the initiation of lung repair, the organization of alveolar exudates, and a shift from a neutrophil- to a lymphocyte-predominant pulmonary infiltrate. As part of the reparative process, type II pneumocytes proliferate along alveolar basement membranes. These specialized epithelial cells synthesize new pulmonary surfactant and differentiate into type I pneumocytes.
Fibrotic Phase While many patients with ARDS recover lung function 3–4 weeks after the initial pulmonary injury, some enter a fibrotic phase that may require long-term support on mechanical ventilators and/or supplemental oxygen. Histologically, the alveolar edema and inflammatory exudates of earlier phases are now converted to extensive alveolar-duct and interstitial fibrosis. Marked disruption of acinar architecture leads to emphysema-like changes, with large bullae. Intimal fibroproliferation in the pulmonary microcirculation causes progressive vascular occlusion and pulmonary hypertension. The physiologic consequences include an increased risk of pneumothorax, reductions in lung compliance, and increased pulmonary dead space. Patients in this late phase experience a substantial burden of excess morbidity. Lung biopsy evidence for pulmonary fibrosis in any phase of ARDS is associated with increased mortality risk.
PROGNOSIS
Mortality Recent mortality estimates for ARDS range from 26% to 44%. There is substantial variability, but a trend toward improved ARDS outcomes appears evident. Of interest, mortality in ARDS is largely attributable to nonpulmonary causes, with sepsis and nonpulmonary organ failure accounting for >80% of deaths. Thus, improvement in survival is likely secondary to advances in the care of septic/infected patients and those with multiple organ failure (Chap. 321).
The major risk factors for ARDS mortality are nonpulmonary. Advanced age is an important risk factor. Patients >75 years of age have a substantially higher mortality risk (~60%) than those <45 (~20%). Moreover, patients >60 years of age with ARDS and sepsis have a threefold higher mortality risk than those <60. Other risk factors include preexisting organ dysfunction from chronic medical illness—in particular, chronic liver disease, cirrhosis, chronic alcohol abuse, chronic immunosuppression, sepsis, chronic renal disease, failure of any nonpulmonary organ, and increased APACHE III scores (Chap. 321). Patients with ARDS arising from direct lung injury (including pneumonia, pulmonary contusion, and aspiration; Table 322-1) are nearly twice as likely to die as those with indirect causes of lung injury, while surgical and trauma patients with ARDS—especially those without direct lung injury—have a higher survival rate than other ARDS patients.
An early (within 24 h of presentation) elevation in pulmonary dead space (>0.60) and severe arterial hypoxemia (PaO2/FIO2, <100 mmHg) predict increased mortality risk from ARDS; however, there is surprisingly little additional value in predicting ARDS mortality from other measures of the severity of lung injury, including the level of PEEP (≥10 cm H2O), respiratory system compliance (≤40 mL/cm H2O), the extent of alveolar infiltrates on chest radiography, and the corrected expired volume per minute (≥10 L/min).
Functional Recovery in ARDS Survivors While it is common for patients with ARDS to experience prolonged respiratory failure and remain dependent on mechanical ventilation for survival, it is a testament to the resolving powers of the lung that the majority of patients recover nearly normal lung function. Patients usually recover maximal lung function within 6 months. One year after endotracheal extubation, more than one-third of ARDS survivors have normal spirometry values and diffusion capacity. Most of the remaining patients have only mild abnormalities in pulmonary function. Unlike mortality risk, recovery of lung function is strongly associated with the extent of lung injury in early ARDS. Low static respiratory compliance, high levels of required PEEP, longer durations of mechanical ventilation, and high lung injury scores are all associated with less recovery of pulmonary function. Of note, when physical function is assessed 5 years after ARDS, exercise limitation and decreased physical quality of life are often documented despite normal or nearly normal pulmonary function. When caring for ARDS survivors, it is important to be aware of the potential for a substantial burden of psychological problems in patients and family caregivers, including significant rates of depression and posttraumatic stress disorder.
WEBSITES
ARDS Support Center for patient-oriented education: www.ards.org
NHLBI ARDS Clinical Trials information: www.ardsnet.org
ARDS Foundation: www.ardsusa.org
ACKNOWLEDGMENT
The authors acknowledge the contribution to this chapter by the previous author, Dr. Steven D. Shapiro.
323 | Mechanical Ventilatory Support |
MECHANICAL VENTILATORY SUPPORT
Mechanical ventilation is used to assist or replace spontaneous breathing. It is implemented with special devices that can support ventilatory function and improve oxygenation through the application of high-oxygen-content gas and positive pressure. The primary indication for initiation of mechanical ventilation is respiratory failure, of which there are two basic types: (1) hypoxemic, which is present when arterial O2 saturation (SaO2) <90% occurs despite an increased inspired O2 fraction and usually results from ventilation-perfusion mismatch or shunt; and (2) hypercarbic, which is characterized by elevated arterial carbon dioxide partial pressure (PCO2) values (usually >50 mmHg) resulting from conditions that decrease minute ventilation or increase physiologic dead space such that alveolar ventilation is inadequate to meet metabolic demands. When respiratory failure is chronic, neither of the two types is obligatorily treated with mechanical ventilation, but when it is acute, mechanical ventilation may be lifesaving.
INDICATIONS
The most common reasons for instituting mechanical ventilation are acute respiratory failure with hypoxemia (acute respiratory distress syndrome, heart failure with pulmonary edema, pneumonia, sepsis, complications of surgery and trauma), which accounts for ~65% of all ventilated cases, and hypercarbic ventilatory failure—e.g., due to coma (15%), exacerbations of chronic obstructive pulmonary disease (COPD; 13%), and neuromuscular diseases (5%). The primary objectives of mechanical ventilation are to decrease the work of breathing, thus avoiding respiratory muscle fatigue, and to reverse life-threatening hypoxemia and progressive respiratory acidosis.
In some cases, mechanical ventilation is used as an adjunct to other forms of therapy. For example, it is used to reduce cerebral blood flow in patients with increased intracranial pressure. Mechanical ventilation also is used frequently in conjunction with endotracheal intubation for airway protection to prevent aspiration of gastric contents in otherwise unstable patients during gastric lavage for suspected drug overdose or during gastrointestinal endoscopy. In critically ill patients, intubation and mechanical ventilation may be indicated before the performance of essential diagnostic or therapeutic studies if it appears that respiratory failure may occur during those maneuvers.
TYPES OF MECHANICAL VENTILATION
There are two basic methods of mechanical ventilation: noninvasive ventilation (NIV) and invasive (or conventional mechanical) ventilation (MV).
Noninvasive Ventilation NIV has gained acceptance because it is effective in certain conditions, such as acute or chronic respiratory failure, and is associated with fewer complications—namely, pneumonia and tracheolaryngeal trauma. NIV usually is provided with a tight-fitting face mask or nasal mask similar to the masks traditionally used for treatment of sleep apnea. NIV has proved highly effective in patients with respiratory failure arising from acute exacerbations of chronic obstructive pulmonary disease. It is most frequently implemented as bilevel positive airway pressure ventilation or pressure-support ventilation. Both modes, which apply a preset positive pressure during inspiration and a lower pressure during expiration at the mask, are well tolerated by a conscious patient and optimize patient-ventilator synchrony. The major limitation to the widespread application of NIV has been patient intolerance: the tight-fitting mask required for NIV can cause both physical and psychological discomfort. In addition, NIV has had limited success in patients with acute hypoxemic respiratory failure, for whom endotracheal intubation and conventional MV remain the ventilatory method of choice.
The most important group of patients who benefit from a trial of NIV are those with exacerbations of COPD and respiratory acidosis (pH <7.35). Experience from several randomized trials has shown that, in patients with ventilatory failure characterized by blood pH levels between 7.25 and 7.35, NIV is associated with low failure rates (15–20%) and good outcomes (as judged by intubation rate, length of stay in intensive care, and—in some series—mortality rates). In more severely ill patients with a blood pH <7.25, the rate of NIV failure is inversely related to the severity of respiratory acidosis, with higher failure rates as the pH decreases. In patients with milder acidosis (pH >7.35), NIV is not better than conventional treatment that includes controlled oxygen delivery and pharmacotherapy for exacerbations of COPD (systemic glucocorticoids, bronchodilators, and, if needed, antibiotics).
Despite its benign outcomes, NIV is not useful in the majority of cases of respiratory failure and is contraindicated in patients with the conditions listed in Table 323-1. NIV can delay lifesaving ventilatory support in those cases and, in fact, can actually result in aspiration or hypoventilation. Once NIV is initiated, patients should be monitored; a reduction in respiratory frequency and a decrease in the use of accessory muscles (scalene, sternomastoid, and intercostals) are good clinical indicators of adequate therapeutic benefit. Arterial blood gases should be determined at least within hours of the initiation of therapy to ensure that NIV is having the desired effect. Lack of benefit within that time frame should alert the physician to the possible need for conventional MV.
CONTRAINDICATIONS FOR NONINVASIVE VENTILATION |
Conventional Mechanical Ventilation Conventional MV is implemented once a cuffed tube is inserted into the trachea to allow conditioned gas (warmed, oxygenated, and humidified) to be delivered to the airways and lungs at pressures above atmospheric pressure. Care should be taken during intubation to avoid brain-damaging hypoxia. In most cases, the administration of mild sedation may facilitate the procedure. Opiates and benzodiazepines are good choices but can have a deleterious effect on hemodynamics in patients with depressed cardiac function or low systemic vascular resistance. Morphine can promote histamine release from tissue mast cells and may worsen bronchospasm in patients with asthma; fentanyl, sufentanil, and alfentanil are acceptable alternatives. Ketamine may increase systemic arterial pressure and has been associated with hallucinatory responses. The shorter-acting agents etomidate and propofol have been used for both induction and maintenance of anesthesia in ventilated patients because they have fewer adverse hemodynamic effects, but both are significantly more expensive than older agents. Great care must be taken to avoid the use of neuromuscular paralysis during intubation of patients with renal failure, tumor lysis syndrome, crush injuries, medical conditions associated with elevated serum potassium levels, and muscular dystrophy syndromes; in particular, the use of agents whose mechanism of action includes depolarization at the neuromuscular junction, such as succinylcholine chloride, must be avoided.
PRINCIPLES OF MECHANICAL VENTILATION
Once the patient has been intubated, the basic goals of MV are to optimize oxygenation while avoiding ventilator-induced lung injury due to overstretch and collapse/re-recruitment. This concept, known as the “protective ventilatory strategy” (see below and Fig. 323-1) is supported by evidence linking high airway pressures and volumes and overstretching of the lung as well as collapse/re-recruitment to poor clinical outcomes (barotrauma and volume trauma). Although normalization of pH through elimination of CO2 is desirable, the risk of lung damage associated with the large volume and high pressures needed to achieve this goal has led to the acceptance of permissive hypercapnia. This condition is well tolerated when care is taken to avoid excess acidosis by pH buffering.
FIGURE 323-1 Hypothetical pressure-volume curve of the lung in a patient undergoing mechanical ventilation. Alveoli tend to close if the distending pressure falls below the lower inflection point (A), whereas they overstretch if the pressure within them is higher than that of the upper inflection point (B). Collapse and opening of ventilated alveoli are associated with poor outcomes in patients with acute respiratory failure. Protective ventilation (purple shaded area), using a lower tidal volume (6 mL/kg of ideal body weight) and maintaining positive end-expiratory pressure to prevent overstretching and collapse/opening of alveoli, has resulted in improved survival rates among patients receiving mechanical ventilatory support.
MODES OF VENTILATION
Mode refers to the manner in which ventilator breaths are triggered, cycled, and limited. The trigger, either an inspiratory effort or a time-based signal, defines what the ventilator senses to initiate an assisted breath. Cycle refers to the factors that determine the end of inspiration. For example, in volume-cycled ventilation, inspiration ends when a specific tidal volume is delivered. Other types of cycling include pressure cycling and time cycling. The limiting factors are operator-specified values, such as airway pressure, that are monitored by transducers internal to the ventilator circuit throughout the respiratory cycle; if the specified values are exceeded, inspiratory flow is terminated, and the ventilator circuit is vented to atmospheric pressure or the specified pressure at the end of expiration (positive end-expiratory pressure, or PEEP). Most patients are ventilated with assist-control ventilation, intermittent mandatory ventilation, or pressure-support ventilation, with the latter two modes often used simultaneously (Table 323-2).
CHARACTERISTICS OF THE MOST COMMONLY USED FORMS OF MECHANICAL VENTILATION |
Assist-Control Ventilation (ACMV) ACMV is the most widely used mode of ventilation. In this mode, an inspiratory cycle is initiated either by the patient’s inspiratory effort or, if none is detected within a specified time window, by a timer signal within the ventilator. Every breath delivered, whether patient- or timer-triggered, consists of the operator-specified tidal volume. Ventilatory rate is determined either by the patient or by the operator-specified backup rate, whichever is of higher frequency. ACMV is commonly used for initiation of mechanical ventilation because it ensures a backup minute ventilation in the absence of an intact respiratory drive and allows for synchronization of the ventilator cycle with the patient’s inspiratory effort.
Problems can arise when ACMV is used in patients with tachypnea due to nonrespiratory or nonmetabolic factors, such as anxiety, pain, and airway irritation. Respiratory alkalemia may develop and trigger myoclonus or seizures. Dynamic hyperinflation leading to increased intrathoracic pressures (so-called auto-PEEP) may occur if the patient’s respiratory mechanics are such that inadequate time is available for complete exhalation between inspiratory cycles. Auto-PEEP can limit venous return, decrease cardiac output, and increase airway pressures, predisposing to barotrauma.
Intermittent Mandatory Ventilation (IMV) With this mode, the operator sets the number of mandatory breaths of fixed volume to be delivered by the ventilator; between those breaths, the patient can breathe spontaneously. In the most frequently used synchronized mode (SIMV), mandatory breaths are delivered in synchrony with the patient’s inspiratory efforts at a frequency determined by the operator. If the patient fails to initiate a breath, the ventilator delivers a fixed-tidal-volume breath and resets the internal timer for the next inspiratory cycle. SIMV differs from ACMV in that only a preset number of breaths are ventilator-assisted.
SIMV allows patients with an intact respiratory drive to exercise inspiratory muscles between assisted breaths; thus it is useful for both supporting and weaning intubated patients. SIMV may be difficult to use in patients with tachypnea because they may attempt to exhale during the ventilator-programmed inspiratory cycle. Consequently, the airway pressure may exceed the inspiratory pressure limit, the ventilator-assisted breath will be aborted, and minute volume may drop below that programmed by the operator. In this setting, if the tachypnea represents a response to respiratory or metabolic acidosis, a change in ACMV will increase minute ventilation and help normalize the pH while the underlying process is further evaluated and treated.
Pressure-Support Ventilation (PSV) This form of ventilation is patient-triggered, flow-cycled, and pressure-limited. It provides graded assistance and differs from the other two modes in that the operator sets the pressure level (rather than the volume) to augment every spontaneous respiratory effort. The level of pressure is adjusted by observing the patient’s respiratory frequency. During PSV, the inspiration is terminated when inspiratory airflow falls below a certain level; in most ventilators, this flow rate cannot be adjusted by the operator. With PSV, patients receive ventilator assistance only when the ventilator detects an inspiratory effort. PSV is often used in combination with SIMV to ensure volume-cycled backup for patients whose respiratory drive is depressed. PSV is well tolerated by most patients who are being weaned from MV; PSV parameters can be set to provide full ventilatory support and can be withdrawn to load the respiratory muscles gradually.
Other Modes of Ventilation There are other modes of ventilation, each with its own acronym and each with specific modifications of the manner and duration in which pressure is applied to the airway and lungs and of the interaction between the mechanical assistance provided by the ventilator and the patient’s respiratory effort. Although their use in acute respiratory failure is limited, the following modes have been used with varying levels of enthusiasm and adoption.
PRESSURE-CONTROL VENTILATION (PCV) This form of ventilation is time-triggered, time-cycled, and pressure-limited. A specified pressure is imposed at the airway opening throughout inspiration. Since the inspiratory pressure is specified by the operator, tidal volume and inspiratory flow rate are dependent, rather than independent, variables and are not operator-specified. PCV is the preferred mode of ventilation for patients in whom it is desirable to regulate peak airway pressures, such as those with preexisting barotrauma, and for post–thoracic surgery patients, in whom the shear forces across a fresh suture line should be limited. When PCV is used, minute ventilation is altered through changes in rate or in the pressure-control value, with consequent changes in tidal volume.
INVERSE-RATIO VENTILATION (IRV) This mode is a variant of PCV that incorporates the use of a prolonged inspiratory time with the appropriate shortening of the expiratory time. IRV has been used in patients with severe hypoxemic respiratory failure. This approach increases mean distending pressures without increasing peak airway pressures. It is thought to work in conjunction with PEEP to open collapsed alveoli and improve oxygenation. However, no clinical-trial data have shown that IRV improves outcomes.
CONTINUOUS POSITIVE AIRWAY PRESSURE (CPAP) CPAP is not a true support mode of ventilation because all ventilation occurs through the patient’s spontaneous efforts. The ventilator provides fresh gas to the breathing circuit with each inspiration and sets the circuit to a constant, operator-specified pressure. CPAP is used to assess extubation potential in patients who have been effectively weaned and who require little ventilatory support and in patients with intact respiratory system function who require an endotracheal tube for airway protection.
Nonconventional Ventilatory Strategies Several nonconventional strategies have been evaluated for their ability to improve oxygenation and reduce mortality rates in patients with advanced hypoxemic respiratory failure. These strategies include high-frequency oscillatory ventilation (HFOV), airway pressure release ventilation (APRV), extracorporeal membrane oxygenation (ECMO), and partial liquid ventilation (PLV) using perfluorocarbons. Although case reports and small uncontrolled cohort studies have shown benefit, randomized controlled trials have failed to demonstrate consistent improvements in outcome with most of these strategies. A recent randomized trial of ECMO documented positive outcomes, but the technique remains controversial because older studies failed to document positive results. Currently, these approaches should be thought of as “salvage” techniques and considered for patients with hypoxemia refractory to conventional therapy. Prone positioning of patients with refractory hypoxemia has also been explored because, in theory, lying prone should improve ventilation-perfusion matching. Several randomized trials in patients with acute lung injury did not demonstrate a survival advantage with prone positioning despite demonstration of a transient physiologic benefit. The administration of nitric oxide gas, which has bronchodilator and pulmonary vasodilator effects when delivered through the airways and improves arterial oxygenation in many patients with advanced hypoxemic respiratory failure, also failed to improve outcomes in these patients with acute lung injury.
The design of new ventilator modes reflect attempts to improve patient-ventilator synchrony—a major practical issue during MV—by allowing patients to trigger the ventilator with their own effort while also incorporating flow algorithms that terminate the cycles once certain preset criteria are reached; this approach has greatly improved patient comfort. New modes of ventilation that synchronize not only the timing but also the levels of assistance to match the patient’s effort have been developed. Proportional assist ventilation (PAV) and neurally adjusted ventilatory-assist ventilation (NAV) are two modes that are designed to deliver assisted breaths through algorithms incorporating not only pressure, volume, and time but also overall respiratory resistance as well as compliance (in the case of PAV) and neural activation of the diaphragm (in the case of NAV). Although these modes enhance patient-ventilator synchrony, their practical use in the everyday management of patients undergoing MV needs further study.
PROTECTIVE VENTILATORY STRATEGY
Whichever mode of MV is used in acute respiratory failure, the evidence from several important controlled trials indicates that a protective ventilation approach guided by the following principles (and summarized in Fig. 323-1) is safe and offers the best chance of a good outcome: (1) Set a target tidal volume close to 6 mL/kg of ideal body weight. (2) Prevent plateau pressure (static pressure in the airway at the end of inspiration) exceeding 30 cm H2O. (3) Use the lowest possible fraction of inspired oxygen (FIO2) to keep the SaO2 at ≥90%. (4) Adjust the PEEP to maintain alveolar patency while preventing overdistention and closure/reopening. With the application of these techniques, the mortality rate among patients with acute hypoxemic respiratory failure has decreased to ~30% from close to 50% a decade ago.
PATIENT MANAGEMENT
Once the patient has been stabilized with respect to gas exchange, definitive therapy for the underlying process responsible for respiratory failure is initiated. Subsequent modifications in ventilator therapy must be provided in parallel with changes in the patient’s clinical status. As improvement in respiratory function is noted, the first priority is to reduce the level of mechanical ventilatory support. Patients on full ventilatory support should be monitored frequently, with the goal of switching to a mode that allows for weaning as soon as possible. Protocols and guidelines that can be applied by paramedical personnel when physicians are not readily available have proved to be of value in shortening ventilator and intensive care unit (ICU) time, with very good outcomes. Patients whose condition continues to deteriorate after ventilatory support is initiated may require increased O2, PEEP, or one of the alternative modes of ventilation.
GENERAL SUPPORT DURING VENTILATION
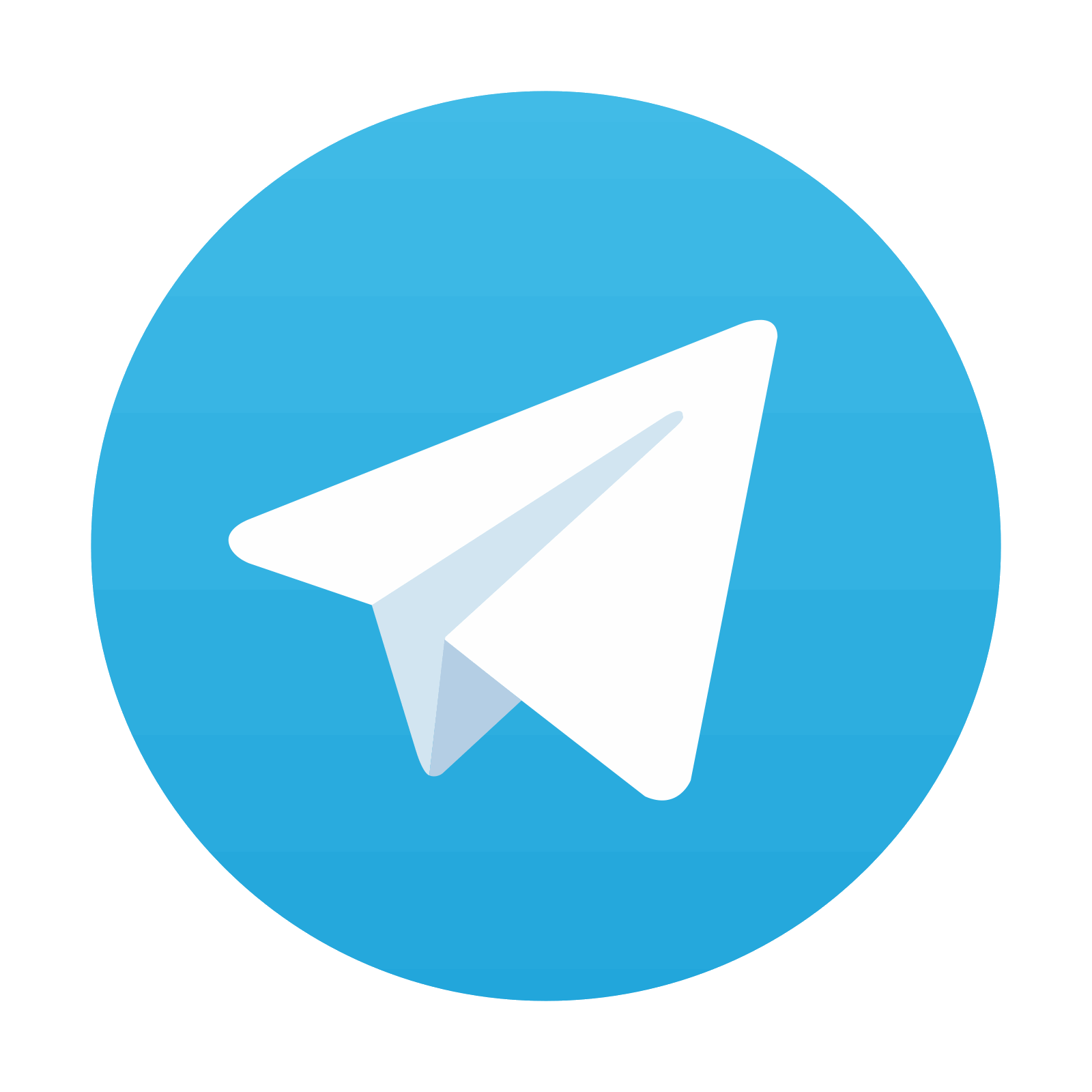
Stay updated, free articles. Join our Telegram channel
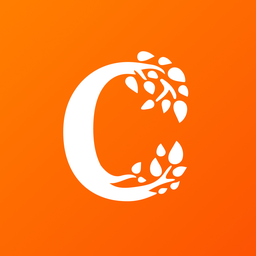
Full access? Get Clinical Tree
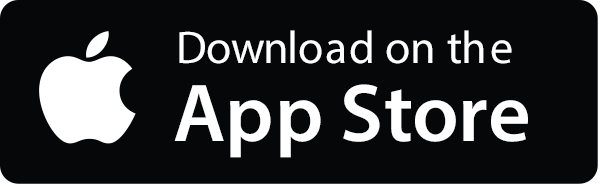
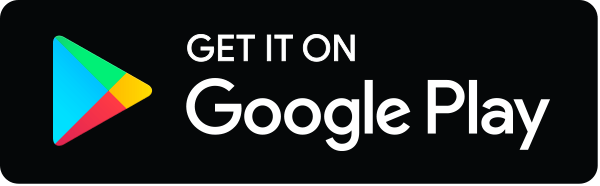