PART 11: Disorders of the Respiratory System
SECTION 1 | DIAGNOSIS OF RESPIRATORY DISORDERS |
305 | Approach to the Patient with Disease of the Respiratory System |
The majority of diseases of the respiratory system fall into one of three major categories: (1) obstructive lung diseases; (2) restrictive disorders; and (3) abnormalities of the vasculature. Obstructive lung diseases are most common and primarily include disorders of the airways, such as asthma, chronic obstructive pulmonary disease (COPD), bronchiectasis, and bronchiolitis. Diseases resulting in restrictive pathophysiology include parenchymal lung diseases, abnormalities of the chest wall and pleura, and neuromuscular disease. Disorders of the pulmonary vasculature include pulmonary embolism, pulmonary hypertension, and pulmonary veno-occlusive disease. Although many specific diseases fall into these major categories, both infective and neoplastic processes can affect the respiratory system and result in myriad pathologic findings, including those listed in the three categories above (Table 305-1).
CATEGORIES OF RESPIRATORY DISEASE |
Disorders can also be grouped according to gas exchange abnormalities, including hypoxemic, hypercarbic, or combined impairment. However, many diseases of the lung do not manifest as gas exchange abnormalities.
As with the evaluation of most patients, the approach to a patient with disease of the respiratory system begins with a thorough history and a focused physical examination. Many patients will subsequently undergo pulmonary function testing, chest imaging, blood and sputum analysis, a variety of serologic or microbiologic studies, and diagnostic procedures, such as bronchoscopy. This stepwise approach is discussed in detail below.
HISTORY
Dyspnea and Cough The cardinal symptoms of respiratory disease are dyspnea and cough (Chaps. 47e and 48). Dyspnea has many causes, some of which are not predominantly due to lung pathology. The words a patient uses to describe shortness of breath can suggest certain etiologies for dyspnea. Patients with obstructive lung disease often complain of “chest tightness” or “inability to get a deep breath,” whereas patients with congestive heart failure more commonly report “air hunger” or a sense of suffocation.
The tempo of onset and the duration of a patient’s dyspnea are likewise helpful in determining the etiology. Acute shortness of breath is usually associated with sudden physiologic changes, such as laryngeal edema, bronchospasm, myocardial infarction, pulmonary embolism, or pneumothorax. Patients with COPD and idiopathic pulmonary fibrosis (IPF) experience a gradual progression of dyspnea on exertion, punctuated by acute exacerbations of shortness of breath. In contrast, most asthmatics have normal breathing the majority of the time with recurrent episodes of dyspnea that are usually associated with specific triggers, such as an upper respiratory tract infection or exposure to allergens.
Specific questioning should focus on factors that incite dyspnea as well as on any intervention that helps resolve the patient’s shortness of breath. Asthma is commonly exacerbated by specific triggers, although this can also be true of COPD. Many patients with lung disease report dyspnea on exertion. Determining the degree of activity that results in shortness of breath gives the clinician a gauge of the patient’s degree of disability. Many patients adapt their level of activity to accommodate progressive limitation. For this reason, it is important, particularly in older patients, to delineate the activities in which they engage and how these activities have changed over time. Dyspnea on exertion is often an early symptom of underlying lung or heart disease and warrants a thorough evaluation.
Cough generally indicates disease of the respiratory system. The clinician should inquire about the duration of the cough, whether or not it is associated with sputum production, and any specific triggers that induce it. Acute cough productive of phlegm is often a symptom of infection of the respiratory system, including processes affecting the upper airway (e.g., sinusitis, tracheitis), the lower airways (e.g., bronchitis, bronchiectasis), and the lung parenchyma (e.g., pneumonia). Both the quantity and quality of the sputum, including whether it is blood-streaked or frankly bloody, should be determined. Hemoptysis warrants an evaluation as delineated in Chap. 48.
Chronic cough (defined as that persisting for >8 weeks) is commonly associated with obstructive lung diseases, particularly asthma and chronic bronchitis, as well as “nonrespiratory” diseases, such as gastroesophageal reflux and postnasal drip. Diffuse parenchymal lung diseases, including IPF, frequently present as a persistent, nonproductive cough. As with dyspnea, all causes of cough are not respiratory in origin, and assessment should encompass a broad differential, including cardiac and gastrointestinal diseases as well as psychogenic causes.
Additional Symptoms Patients with respiratory disease may report wheezing, which is suggestive of airways disease, particularly asthma. Hemoptysis can be a symptom of a variety of lung diseases, including infections of the respiratory tract, bronchogenic carcinoma, and pulmonary embolism. In addition, chest pain or discomfort is often thought to be respiratory in origin. As the lung parenchyma is not innervated with pain fibers, pain in the chest from respiratory disorders usually results from either diseases of the parietal pleura (e.g., pneumothorax) or pulmonary vascular diseases (e.g., pulmonary hypertension). As many diseases of the lung can result in strain on the right side of the heart, patients may also present with symptoms of cor pulmonale, including abdominal bloating or distention and pedal edema (Chap. 279).
Additional History A thorough social history is an essential component of the evaluation of patients with respiratory disease. All patients should be asked about current or previous cigarette smoking, as this exposure is associated with many diseases of the respiratory system, most notably COPD and bronchogenic lung cancer but also a variety of diffuse parenchymal lung diseases (e.g., desquamative interstitial pneumonitis and pulmonary Langerhans cell histiocytosis). For most disorders, longer duration and greater intensity of exposure to cigarette smoke increases the risk of disease. There is growing evidence that “second-hand smoke” is also a risk factor for respiratory tract pathology; for this reason, patients should be asked about parents, spouses, or housemates who smoke. Possible inhalational exposures should be explored, including those at the work place (e.g., asbestos, wood smoke) and those associated with leisure (e.g., excrement from pet birds) (Chap. 311). Travel predisposes to certain infections of the respiratory tract, most notably the risk of tuberculosis. Potential exposure to fungi found in specific geographic regions or climates (e.g., Histoplasma capsulatum) should be explored.
Associated symptoms of fever and chills should raise the suspicion of infective etiologies, both pulmonary and systemic. A comprehensive review of systems may suggest rheumatologic or autoimmune disease presenting with respiratory tract manifestations. Questions should focus on joint pain or swelling, rashes, dry eyes, dry mouth, or constitutional symptoms. In addition, carcinomas from a variety of primary sources commonly metastasize to the lung and cause respiratory symptoms. Finally, therapy for other conditions, including both irradiation and medications, can result in diseases of the chest.
Physical Examination The clinician’s suspicion of respiratory disease often begins with a patient’s vital signs. The respiratory rate is often informative, whether elevated (tachypnea) or depressed (hypopnea). In addition, pulse oximetry should be measured, as many patients with respiratory disease have hypoxemia, either at rest or with exertion. The classic structure of the respiratory examination proceeds through inspection, percussion, palpation, and auscultation as described below. Often, however, auscultatory findings will lead the clinician to perform further percussion or palpation in order to clarify these findings.
The first step of the physical examination is inspection. Patients with respiratory disease may be in distress, often using accessory muscles of respiration to breathe. Severe kyphoscoliosis can result in restrictive pathophysiology. Inability to complete a sentence in conversation is generally a sign of severe impairment and should result in an expedited evaluation of the patient.
Percussion of the chest is used to establish diaphragm excursion and lung size. In the setting of decreased breath sounds, percussion is used to distinguish between pleural effusions (dull to percussion) and pneumothorax (hyper-resonant note).
The role of palpation is limited in the respiratory examination. Palpation can demonstrate subcutaneous air in the setting of barotrauma. It can also be used as an adjunctive assessment to determine whether an area of decreased breath sounds is due to consolidation (increased tactile fremitus) or a pleural effusion (decreased tactile fremitus).
The majority of the manifestations of respiratory disease present as abnormalities of auscultation. Wheezes are a manifestation of airway obstruction. While most commonly a sign of asthma, peribronchial edema in the setting of congestive heart failure can also result in diffuse wheezes, as can any other process that causes narrowing of small airways. For this reason, clinicians must take care not to attribute all wheezing to asthma.
Rhonchi are a manifestation of obstruction of medium-sized airways, most often with secretions. In the acute setting, this manifestation may be a sign of viral or bacterial bronchitis. Chronic rhonchi suggest bronchiectasis or COPD. Stridor, a high-pitched, focal inspiratory wheeze, usually heard over the neck, is a manifestation of upper airway obstruction and should prompt expedited evaluation of the patient, as it can precede complete upper airway obstruction and respiratory failure.
Crackles, or rales, are commonly a sign of alveolar disease. A variety of processes that fill the alveoli with fluid may result in crackles. Pneumonia can cause focal crackles. Pulmonary edema is associated with crackles, generally more prominent at the bases. Interestingly, diseases that result in fibrosis of the interstitium (e.g., IPF) also result in crackles often sounding like Velcro being ripped apart. Although some clinicians make a distinction between “wet” and “dry” crackles, this distinction has not been shown to be a reliable way to differentiate among etiologies of respiratory disease.
One way to help distinguish between crackles associated with alveolar fluid and those associated with interstitial fibrosis is to assess for egophony. Egophony is the auscultation of the sound “AH” instead of “EEE” when a patient phonates “EEE.” This change in note is due to abnormal sound transmission through consolidated parenchyma and is present in pneumonia but not in IPF. Similarly, areas of alveolar filling have increased whispered pectoriloquy as well as transmission of larger-airway sounds (i.e., bronchial breath sounds in a lung zone where vesicular breath sounds are expected).
The lack or diminution of breath sounds can also help determine the etiology of respiratory disease. Patients with emphysema often have a quiet chest with diffusely decreased breath sounds. A pneumothorax or pleural effusion may present with an area of absent breath sounds.
Other Systems Pedal edema, if symmetric, may suggest cor pulmonale; if asymmetric, it may be due to deep venous thrombosis and associated pulmonary embolism. Jugular venous distention may also be a sign of volume overload associated with right heart failure. Pulsus paradoxus is an ominous sign in a patient with obstructive lung disease, as it is associated with significant negative intrathoracic (pleural) pressures required for ventilation and impending respiratory failure.
As stated earlier, rheumatologic disease may manifest primarily as lung disease. Owing to this association, particular attention should be paid to joint and skin examination. Clubbing can be found in many lung diseases, including cystic fibrosis, IPF, and lung cancer. Cyanosis is seen in hypoxemic respiratory disorders that result in >5 g of deoxygenated hemoglobin/dL.
DIAGNOSTIC EVALUATION
The sequence of studies is dictated by the clinician’s differential diagnosis, as determined by the history and physical examination. Acute respiratory symptoms are often evaluated with multiple tests performed at the same time in order to diagnose any life-threatening diseases rapidly (e.g., pulmonary embolism or multilobar pneumonia). In contrast, chronic dyspnea and cough can be evaluated in a more protracted, stepwise fashion.
Pulmonary Function Testing (See also Chap. 307) The initial pulmonary function test obtained is spirometry. This study is an effort-dependent test used to assess for obstructive pathophysiology as seen in asthma, COPD, and bronchiectasis. A diminished-forced expiratory volume in 1 sec (FEV1)/forced vital capacity (FVC) (often defined as <70% of the predicted value) is diagnostic of obstruction. In addition to measuring FEV1 and FVC, the clinician should examine the flow-volume loop (which is effort-independent). A plateau of the inspiratory and expiratory curves suggests large-airway obstruction in extrathoracic and intrathoracic locations, respectively.
Spirometry with symmetric decreases in FEV1 and FVC warrants further testing, including measurement of lung volumes and the diffusion capacity of the lung for carbon monoxide (DLCO). A total lung capacity <80% of the predicted value for a patient’s age, race, sex, and height defines restrictive pathophysiology. Restriction can result from parenchymal disease, neuromuscular weakness, or chest wall or pleural diseases. Restriction with impaired gas exchange, as indicated by a decreased DLCO, suggests parenchymal lung disease. Additional testing, such as measurements of maximal expiratory pressure and maximal inspiratory pressure, can help diagnose neuromuscular weakness. Normal spirometry, normal lung volumes, and a low DLCO should prompt further evaluation for pulmonary vascular disease.
Arterial blood gas testing is often helpful in assessing respiratory disease. Hypoxemia, while usually apparent with pulse oximetry, can be further evaluated with the measurement of arterial PO2 and the calculation of an alveolar gas and arterial blood oxygen tension difference ([A–a]DO2). Patients with diseases that cause ventilation-perfusion mismatch or shunt physiology have an increased (A–a) DO2 at rest. Arterial blood gas testing also allows the measurement of arterial PCO2. Hypercarbia can accompany severe airway obstruction (e.g., COPD) or progressive restrictive physiology, as in patients with neuromuscular weakness.
Chest Imaging (see Chap. 308e) Most patients with disease of the respiratory system undergo imaging of the chest as part of the initial evaluation. Clinicians should generally begin with a plain chest radiograph, preferably posterior-anterior and lateral films. Several findings, including opacities of the parenchyma, blunting of the costophrenic angles, mass lesions, and volume loss, can be very helpful in determining an etiology. However, many diseases of the respiratory system, particularly those of the airways and pulmonary vasculature, are associated with a normal chest radiograph.
CT of the chest is often performed subsequently and allows better delineation of parenchymal processes, pleural disease, masses or nodules, and large airways. If the test includes administration of contrast, the pulmonary vasculature can be assessed with particular utility for determination of pulmonary emboli. Intravenous contrast also allows lymph nodes to be delineated in greater detail.
FURTHER STUDIES
Depending on the clinician’s suspicion, a variety of other studies may be done. Concern about large-airway lesions may warrant bronchoscopy. This procedure may also be used to sample the alveolar space with bronchoalveolar lavage or to obtain nonsurgical lung biopsies. Blood testing may include assessment for hypercoagulable states in the setting of pulmonary vascular disease, serologic testing for infectious or rheumatologic disease, or assessment of inflammatory markers or leukocyte counts (e.g., eosinophils). Sputum evaluation for malignant cells or microorganisms may be appropriate. An echocardiogram to assess right- and left-sided heart function is often obtained. Finally, at times, a surgical lung biopsy is needed to diagnose certain diseases of the respiratory system. All of these studies will be guided by the preceding history, physical examination, pulmonary function testing, and chest imaging.
306e | Disturbances of Respiratory Function |
The primary functions of the respiratory system—to oxygenate blood and eliminate carbon dioxide—require virtual contact between blood and fresh air, which facilitates diffusion of respiratory gases between blood and gas. This process occurs in the lung alveoli, where blood flowing through alveolar wall capillaries is separated from alveolar gas by an extremely thin membrane of flattened endothelial and epithelial cells, across which respiratory gases diffuse and equilibrate. Blood flow through the lung is unidirectional via a continuous vascular path, along which venous blood absorbs oxygen from and loses CO2 to inspired gas. The path for airflow, in contrast, reaches a dead end at the alveolar walls; thus the alveolar space must be ventilated tidally, with inflow of fresh gas and outflow of alveolar gas alternating periodically at the respiratory rate (RR). To provide an enormous alveolar surface area (typically 70 m2) for blood-gas diffusion within the modest volume of a thoracic cavity (typically 7 L), nature has distributed both blood flow and ventilation among millions of tiny alveoli through multigenerational branching of both pulmonary arteries and bronchial airways. As a consequence of variations in tube lengths and calibers along these pathways as well as the effects of gravity, tidal pressure fluctuations, and anatomic constraints from the chest wall, the alveoli vary in their relative ventilations and perfusions. Not surprisingly, for the lung to be most efficient in exchanging gas, the fresh gas ventilation of a given alveolus must be matched to its perfusion.
For the respiratory system to succeed in oxygenating blood and eliminating CO2, it must be able to ventilate the lung tidally and thus to freshen alveolar gas; it must provide for perfusion of the individual alveolus in a manner proportional to its ventilation; and it must allow adequate diffusion of respiratory gases between alveolar gas and capillary blood. Furthermore, it must accommodate severalfold increases in the demand for oxygen uptake or CO2 elimination imposed by metabolic needs or acid-base derangement. Given these multiple requirements for normal operation, it is not surprising that many diseases disturb respiratory function. This chapter considers in some detail the physiologic determinants of lung ventilation and perfusion, elucidates how the matching distributions of these processes and rapid gas diffusion allow normal gas exchange, and discusses how common diseases derange these normal functions, thereby impairing gas exchange—or at least increasing the work required by the respiratory muscles or heart to maintain adequate respiratory function.
VENTILATION
It is useful to think about the respiratory system as three independently functioning components: the lung, including its airways; the neuromuscular system; and the chest wall, which includes everything that is not lung or active neuromuscular system. Accordingly, the mass of the respiratory muscles is part of the chest wall, while the force these muscles generate is part of the neuromuscular system; the abdomen (especially an obese abdomen) and the heart (especially an enlarged heart) are, for these purposes, part of the chest wall. Each of these three components has mechanical properties that relate to its enclosed volume (or—in the case of the neuromuscular system—the respiratory system volume at which it is operating) and to the rate of change of its volume (i.e., flow).
Volume-Related Mechanical Properties—Statics Figure 306e-1 shows the volume-related properties of each component of the respiratory system. Due both to surface tension at the air-liquid interface between alveolar wall lining fluid and alveolar gas and to elastic recoil of the lung tissue itself, the lung requires a positive transmural pressure difference between alveolar gas and its pleural surface to stay inflated; this difference is called the elastic recoil pressure of the lung, and it increases with lung volume. The lung becomes rather stiff at high volumes, so that relatively small volume changes are accompanied by large changes in transpulmonary pressure; in contrast, the lung is compliant at lower volumes, including those at which tidal breathing normally occurs. At zero inflation pressure, even normal lungs retain some air in the alveoli because the small peripheral airways are tethered open by radially outward pull from inflated lung parenchyma attached to adventitia; as the lung deflates during exhalation, those small airways are pulled open progressively less, and eventually they close, trapping some gas in the alveoli. This effect can be exaggerated with age and especially with obstructive airway diseases, resulting in gas trapping at quite large lung volumes.
FIGURE 306e-1 Pressure-volume curves of the isolated lung, isolated chest wall, combined respiratory system, inspiratory muscles, and expiratory muscles. FRC, functional residual capacity; RV, residual volume; TLC, total lung capacity.
The elastic behavior of the passive chest wall (i.e., in the absence of neuromuscular activation) differs markedly from that of the lung. Whereas the lung tends toward full deflation with no distending (transmural) pressure, the chest wall encloses a large volume when pleural pressure equals body surface (atmospheric) pressure. Furthermore, the chest wall is compliant at high enclosed volumes, readily expanding even further in response to increases in transmural pressure. The chest wall also remains compliant at small negative transmural pressures (i.e., when pleural pressure falls slightly below atmospheric pressure), but as the volume enclosed by the chest wall becomes quite small in response to large negative transmural pressures, the passive chest wall becomes stiff due to squeezing together of ribs and intercostal muscles, diaphragm stretch, displacement of abdominal contents, and straining of ligaments and bony articulations. Under normal circumstances, the lung and the passive chest wall enclose essentially the same volume, the only difference being the volumes of the pleural fluid and of the lung parenchyma (both quite small). For this reason and because the lung and chest wall function in mechanical series, the pressure required to displace the passive respiratory system (lungs plus chest wall) at any volume is simply the sum of the elastic recoil pressure of the lungs and the transmural pressure across the chest wall. When plotted against respiratory system volume, this relationship assumes a sigmoid shape, exhibiting stiffness at high lung volumes (imparted by the lung), stiffness at low lung volumes (imparted by the chest wall or sometimes by airway closure), and compliance in the middle range of lung volumes. In addition, a passive resting point of the respiratory system is attained when alveolar gas pressure equals body surface pressure (i.e., when the transrespiratory system pressure is zero). At this volume (called the functional residual capacity [FRC]), the outward recoil of the chest wall is balanced exactly by the inward recoil of the lung. As these recoils are transmitted through the pleural fluid, the lung is pulled both outward and inward simultaneously at FRC, and thus its pressure falls below atmospheric pressure (typically, –5 cmH2O).
The normal passive respiratory system would equilibrate at the FRC and remain there were it not for the actions of respiratory muscles. The inspiratory muscles act on the chest wall to generate the equivalent of positive pressure across the lungs and passive chest wall, while the expiratory muscles generate the equivalent of negative transrespiratory pressure. The maximal pressures these sets of muscles can generate vary with the lung volume at which they operate. This variation is due to length-tension relationships in striated muscle sarcomeres and to changes in mechanical advantage as the angles of insertion change with lung volume (Fig. 306e-1). Nonetheless, under normal conditions, the respiratory muscles are substantially “overpowered” for their roles and generate more than adequate force to drive the respiratory system to its stiffness extremes, as determined by the lung (total lung capacity [TLC]) or by chest wall or airway closure (residual volume [RV]); the airway closure always prevents the adult lung from emptying completely under normal circumstances. The excursion between full and minimal lung inflation is called vital capacity (VC; Fig. 306e-2) and is readily seen to be the difference between volumes at two unrelated stiffness extremes—one determined by the lung (TLC) and the other by the chest wall or airways (RV). Thus, although VC is easy to measure (see below), it provides little information about the intrinsic properties of the respiratory system. As will become clear, it is much more useful for the clinician to consider TLC and RV individually.
FIGURE 306e-2 Spirogram demonstrating a slow vital capacity maneuver and various lung volumes.
Flow-Related Mechanical Properties—Dynamics The passive chest wall and active neuromuscular system do exhibit mechanical behaviors related to the rate of change of volume, but these behaviors become quantitatively important only at markedly supraphysiologic breathing frequencies (e.g., during high-frequency mechanical ventilation) and thus will not be addressed here. In contrast, the dynamic airflow properties of the lung substantially affect its ability to ventilate and contribute importantly to the work of breathing, and these properties are often deranged by disease. Understanding dynamic airflow properties is therefore worthwhile.
As with the flow of any fluid (gas or liquid) in any tube, maintenance of airflow within the pulmonary airways requires a pressure gradient that falls along the direction of flow, the magnitude of which is determined by the flow rate and the frictional resistance to flow. During quiet tidal breathing, the pressure gradients driving inspiratory or expiratory flow are small owing to the very low frictional resistance of normal pulmonary airways (Raw, normally <2 cmH2O/L per second). However, during rapid exhalation, another phenomenon reduces flow below that which would have been expected if frictional resistance were the only impediment to flow. This phenomenon is called dynamic airflow limitation, and it occurs because the bronchial airways through which air is exhaled are collapsible rather than rigid (Fig. 306e-3). An important anatomic feature of the pulmonary airways is its treelike branching structure. While the individual airways in each successive generation, from most proximal (trachea) to most distal (respiratory bronchioles), are smaller than those of the parent generation, their number increases exponentially such that the summed cross-sectional area of the airways becomes very large toward the lung periphery. Because flow (volume/time) is constant along the airway tree, the velocity of airflow (flow/summed cross-sectional area) is much greater in the central airways than in the peripheral airways. During exhalation, gas leaving the alveoli must therefore gain velocity as it proceeds toward the mouth. The energy required for this “convective” acceleration is drawn from the component of gas energy manifested as its local pressure, which reduces intraluminal gas pressure, airway transmural pressure, airway size (Fig. 306e-3), and flow. This is the Bernoulli effect, the same effect that keeps an airplane airborne, generating a lifting force by decreasing pressure above the curved upper surface of the wing due to acceleration of air flowing over the wing. If an individual tries to exhale more forcefully, the local velocity increases further and reduces airway size further, resulting in no net increase in flow. Under these circumstances, flow has reached its maximum possible value, or its flow limit. Lungs normally exhibit such dynamic airflow limitation. This limitation can be assessed by spirometry, in which an individual inhales fully to TLC and then forcibly exhales to RV. One useful spirometric measure is the volume of air exhaled during the first second of expiration (FEV1), as discussed later. Maximal expiratory flow at any lung volume is determined by gas density, airway cross-section and distensibility, elastic recoil pressure of the lung, and frictional pressure loss to the flow-limiting airway site. Under normal conditions, maximal expiratory flow falls with lung volume (Fig. 306e-4), primarily because of the dependence of lung recoil pressure on lung volume (Fig. 306e-1). In pulmonary fibrosis, lung recoil pressure is increased at any lung volume, and thus the maximal expiratory flow is elevated when considered in relation to lung volume. Conversely, in emphysema, lung recoil pressure is reduced; this reduction is a principal mechanism by which maximal expiratory flows fall. Diseases that narrow the airway lumen at any transmural pressure (e.g., asthma or chronic bronchitis) or that cause excessive airway collapsibility (e.g., tracheomalacia) also reduce maximal expiratory flow.
FIGURE 306e-3 Luminal area versus transmural pressure relationship. Transmural pressure represents the pressure difference across the airway wall from inside to outside.
The Bernoulli effect also applies during inspiration, but the more negative pleural pressures during inspiration lower the pressure outside of airways, thereby increasing transmural pressure and promoting airway expansion. Thus inspiratory airflow limitation seldom occurs due to diffuse pulmonary airway disease. Conversely, extrathoracic airway narrowing (e.g., due to a tracheal adenoma or post-tracheostomy stricture) can lead to inspiratory airflow limitation (Fig. 306e-4).
FIGURE 306e-4 Flow-volume loops. A. Normal. B. Airflow obstruction. C. Fixed central airway obstruction. RV, residual volume; TLC, total lung capacity.
The Work of Breathing In health, the elastic (volume change–related) and dynamic (flow-related) loads that must be overcome to ventilate the lungs at rest are small, and the work required of the respiratory muscles is minimal. However, the work of breathing can increase considerably due to a metabolic requirement for substantially increased ventilation, an abnormally increased mechanical load, or both. As discussed below, the rate of ventilation is primarily set by the need to eliminate carbon dioxide, and thus ventilation increases during exercise (sometimes by more than twentyfold) and during metabolic acidosis as a compensatory response. Naturally, the work rate required to overcome the elasticity of the respiratory system increases with both the depth and the frequency of tidal breaths, while the work required to overcome the dynamic load increases with total ventilation. A modest increase of ventilation is most efficiently achieved by increasing tidal volume but not respiratory rate, which is the normal ventilatory response to lower-level exercise. At high levels of exercise, deep breathing persists, but respiratory rate also increases. The pattern chosen by the respiratory controller minimizes the work of breathing.
The work of breathing also increases when disease reduces the compliance of the respiratory system or increases the resistance to airflow. The former occurs commonly in diseases of the lung parenchyma (interstitial processes or fibrosis, alveolar filling diseases such as pulmonary edema or pneumonia, or substantial lung resection), and the latter occurs in obstructive airway diseases such as asthma, chronic bronchitis, emphysema, and cystic fibrosis. Furthermore, severe airflow obstruction can functionally reduce the compliance of the respiratory system by leading to dynamic hyperinflation. In this scenario, expiratory flows slowed by the obstructive airways disease may be insufficient to allow complete exhalation during the expiratory phase of tidal breathing; as a result, the “functional residual capacity” from which the next breath is inhaled is greater than the static FRC. With repetition of incomplete exhalations of each tidal breath, the operating FRC becomes dynamically elevated, sometimes to a level that approaches TLC. At these high lung volumes, the respiratory system is much less compliant than at normal breathing volumes, and thus the elastic work of each tidal breath is also increased. The dynamic pulmonary hyperinflation that accompanies severe airflow obstruction causes patients to sense difficulty in inhaling—even though the root cause of this pathophysiologic abnormality is expiratory airflow obstruction.
Adequacy of Ventilation As noted above, the respiratory control system that sets the rate of ventilation responds to chemical signals, including arterial CO2 and oxygen tensions and blood pH, and to volitional needs, such as the need to inhale deeply before playing a long phrase on the trumpet. Disturbances in ventilation are discussed in Chap. 318. The focus of this chapter is on the relationship between ventilation of the lung and CO2 elimination.
At the end of each tidal exhalation, the conducting airways are filled with alveolar gas that had not reached the mouth when expiratory flow stopped. During the ensuing inhalation, fresh gas immediately enters the airway tree at the mouth, but the gas first entering the alveoli at the start of inhalation is that same alveolar gas in the conducting airways that had just left the alveoli. Accordingly, fresh gas does not enter the alveoli until the volume of the conducting airways has been inspired. This volume is called the anatomic dead space (VD). Quiet breathing with tidal volumes smaller than the anatomic dead space introduces no fresh gas into the alveoli at all; only that part of the inspired tidal volume (VT) that is greater than the VD introduces fresh gas into the alveoli. The dead space can be further increased functionally if some of the inspired tidal volume is delivered to a part of the lung that receives no pulmonary blood flow and thus cannot contribute to gas exchange (e.g., the portion of the lung distal to a large pulmonary embolus). In this situation, exhaled minute ventilation (E = VT × RR) includes a component of dead space ventilation (
D = VD × RR) and a component of fresh gas alveolar ventilation (
A = [VT – VD] × RR). CO2 elimination from the alveoli is equal to
A times the difference in CO2 fraction between inspired air (essentially zero) and alveolar gas (typically ~5.6% after correction for humidification of inspired air, corresponding to 40 mmHg). In the steady state, the alveolar fraction of CO2 is equal to metabolic CO2 production divided by alveolar ventilation. Because, as discussed below, alveolar and arterial CO2 tensions are equal, and because the respiratory controller normally strives to maintain arterial PCO2 (PaCO2) at ~40 mmHg, the adequacy of alveolar ventilation is reflected in PaCO2. If the PaCO2 falls much below 40 mmHg, alveolar hyperventilation is present; if the PaCO2 exceeds 40 mmHg, then alveolar hypoventilation is present. Ventilatory failure is characterized by extreme alveolar hypoventilation.
As a consequence of oxygen uptake of alveolar gas into capillary blood, alveolar oxygen tension falls below that of inspired gas. The rate of oxygen uptake (determined by the body’s metabolic oxygen consumption) is related to the average rate of metabolic CO2 production, and their ratio—the “respiratory quotient” (R = CO2/
O2)—depends largely on the fuel being metabolized. For a typical American diet, R is usually around 0.85, and more oxygen is absorbed than CO2 is excreted. Together, these phenomena allow the estimation of alveolar oxygen tension, according to the following relationship, known as the alveolar gas equation:
PAO2 = FIO2 × (Pbar – PH2O) – PACO2/R
The alveolar gas equation also highlights the influences of inspired oxygen fraction (FIO2), barometric pressure (Pbar), and vapor pressure of water (PH2O = 47 mmHg at 37°C) in addition to alveolar ventilation (which sets PACO2) in determining PAO2. An implication of the alveolar gas equation is that severe arterial hypoxemia rarely occurs as a pure consequence of alveolar hypoventilation at sea level while an individual is breathing air. The potential for alveolar hypoventilation to induce severe hypoxemia with otherwise normal lungs increases as Pbar falls with increasing altitude.
GAS EXCHANGE
Diffusion For oxygen to be delivered to the peripheral tissues, it must pass from alveolar gas into alveolar capillary blood by diffusing through alveolar membrane. The aggregate alveolar membrane is highly optimized for this process, with a very large surface area and minimal thickness. Diffusion through the alveolar membrane is so efficient in the human lung that in most circumstances a red blood cell’s hemoglobin becomes fully oxygen saturated by the time the cell has traveled just one-third the length of the alveolar capillary. Thus the uptake of alveolar oxygen is ordinarily limited by the amount of blood transiting the alveolar capillaries rather than by the rapidity with which oxygen can diffuse across the membrane; consequently, oxygen uptake from the lung is said to be “perfusion limited.” CO2 also equilibrates rapidly across the alveolar membrane. Therefore, the oxygen and CO2 tensions in capillary blood leaving a normal alveolus are essentially equal to those in alveolar gas. Only in rare circumstances (e.g., at high altitude or in high-performance athletes exerting maximal effort) is oxygen uptake from normal lungs diffusion limited. Diffusion limitation can also occur in interstitial lung disease if substantially thickened alveolar walls remain perfused.
Ventilation/Perfusion Heterogeneity As noted above, for gas exchange to be most efficient, ventilation to each individual alveolus (among the millions of alveoli) should match perfusion to its accompanying capillaries. Because of the differential effects of gravity on lung mechanics and blood flow throughout the lung and because of differences in airway and vascular architecture among various respiratory paths, there is minor ventilation/perfusion heterogeneity even in the normal lung; however, /
heterogeneity can be particularly marked in disease. Two extreme examples are (1) ventilation of unperfused lung distal to a pulmonary embolus, in which ventilation of the physiologic dead space is “wasted” in the sense that it does not contribute to gas exchange; and (2) perfusion of nonventilated lung (a “shunt”), which allows venous blood to pass through the lung unaltered. When mixed with fully oxygenated blood leaving other well-ventilated lung units, shunted venous blood disproportionately lowers the mixed arterial PaO2 as a result of the nonlinear oxygen content versus PO2 relationship of hemoglobin (Fig. 306e-5). Furthermore, the resulting arterial hypoxemia is refractory to supplemental inspired oxygen. The reason is that (1) raising the inspired FIO2 has no effect on alveolar gas tensions in nonventilated alveoli and (2) while raising inspired FIO2 does increase PACO2 in ventilated alveoli, the oxygen content of blood exiting ventilated units increases only slightly, as hemoglobin will already have been nearly fully saturated and the solubility of oxygen in plasma is quite small.
FIGURE 306e-5 Influence of air versus oxygen breathing on mixed arterial oxygenation in shunt and ventilation/perfusion heterogeneity. Partial pressure of oxygen (mmHg) and oxygen saturations are shown for mixed venous blood, for end capillary blood (normal versus affected alveoli), and for mixed arterial blood. FIO2, fraction of inspired oxygen; /
, ventilation/perfusion.
A more common occurrence than the two extreme examples given above is a widening of the distribution of ventilation/perfusion ratios; such /
heterogeneity is a common consequence of lung disease. In this circumstance, perfusion of relatively underventilated alveoli results in the incomplete oxygenation of exiting blood. When mixed with well-oxygenated blood leaving higher
/
regions, this partially reoxygenated blood disproportionately lowers arterial PaO2, although to a lesser extent than does a similar perfusion fraction of blood leaving regions of pure shunt. In addition, in contrast to shunt regions, inhalation of supplemental oxygen does raise the PAO2, even in relatively underventilated low
/
regions, and so the arterial hypoxemia induced by
/
heterogeneity is typically responsive to oxygen therapy (Fig. 306e-5).
In sum, arterial hypoxemia can be caused by substantial reduction of inspired oxygen tension; by severe alveolar hypoventilation; by perfusion of relatively underventilated (low /
) or completely unventilated (shunt) lung regions; and, in unusual circumstances, by limitation of gas diffusion.
PATHOPHYSIOLOGY
Although many diseases injure the respiratory system, this system responds to injury in relatively few ways. For this reason, the pattern of physiologic abnormalities may or may not provide sufficient information by which to discriminate among conditions.
Figure 306e-6 lists abnormalities in pulmonary function testing that are typically found in a number of common respiratory disorders and highlights the simultaneous occurrence of multiple physiologic abnormalities. The coexistence of some of these respiratory disorders results in more complex superposition of these abnormalities. Methods to measure respiratory system function clinically are described later in this chapter.
FIGURE 306e-6 Common abnormalities of pulmonary function (see text). Pulmonary function values are expressed as a percentage of normal predicted values, except for Raw, which is expressed as cmH2O/L per sec (normal, <2 cmH2O/L per second). The figures at the bottom of each column show the typical configuration of flow-volume loops in each condition, including the flow-volume relationship during tidal breathing. b.d., bronchodilator; DLCO, diffusion capacity of lung for carbon monoxide; FEV1, forced expiratory volume in 1 sec; FRC, functional residual capacity; FVC, forced vital capacity; Raw, airways resistance; RV, residual volume; TLC, total lung capacity.
Ventilatory Restriction Due to Increased Elastic Recoil—Example: Idiopathic Pulmonary Fibrosis Idiopathic pulmonary fibrosis raises lung recoil at all lung volumes, thereby lowering TLC, FRC, and RV as well as forced vital capacity (FVC). Maximal expiratory flows are also reduced from normal values but are elevated when considered in relation to lung volumes. Increased flow occurs both because the increased lung recoil drives greater maximal flow at any lung volume and because airway diameters are relatively increased due to greater radially outward traction exerted on bronchi by the stiff lung parenchyma. For the same reason, airway resistance is also normal. Destruction of the pulmonary capillaries by the fibrotic process results in a marked reduction in diffusing capacity (see below). Oxygenation is often severely reduced by persistent perfusion of alveolar units that are relatively underventilated due to fibrosis of nearby (and mechanically linked) lung. The flow-volume loop (see below) looks like a miniature version of a normal loop but is shifted toward lower absolute lung volumes and displays maximal expiratory flows that are increased for any given volume over the normal tracing.
Ventilatory Restriction Due to Chest Wall Abnormality—Example: Moderate Obesity As the size of the average American continues to increase, this pattern may become the most common of pulmonary function abnormalities. In moderate obesity, the outward recoil of the chest wall is blunted by the weight of chest wall fat and the space occupied by intraabdominal fat. In this situation, preserved inward recoil of the lung overbalances the reduced outward recoil of the chest wall, and FRC falls. Because respiratory muscle strength and lung recoil remain normal, TLC is typically unchanged (although it may fall in massive obesity) and RV is normal (but may be reduced in massive obesity). Mild hypoxemia may be present due to perfusion of alveolar units that are poorly ventilated because of airway closure in dependent portions of the lung during breathing near the reduced FRC. Flows remain normal, as does the diffusion capacity of the lung for carbon monoxide (DLCO), unless obstructive sleep apnea (which often accompanies obesity) and associated chronic intermittent hypoxemia have induced pulmonary arterial hypertension, in which case DLCO may be low.
Ventilatory Restriction Due to Reduced Muscle Strength—Example: Myasthenia Gravis In this circumstance, FRC remains normal, as both lung recoil and passive chest wall recoil are normal. However, TLC is low and RV is elevated because respiratory muscle strength is insufficient to push the passive respiratory system fully toward either volume extreme. Caught between the low TLC and the elevated RV, FVC and FEV1 are reduced as “innocent bystanders.” As airway size and lung vasculature are unaffected, both Raw and DLCO are normal. Oxygenation is normal unless weakness becomes so severe that the patient has insufficient strength to reopen collapsed alveoli during sighs, with resulting atelectasis.
Airflow Obstruction Due to Decreased Airway Diameter—Example: Acute Asthma During an episode of acute asthma, luminal narrowing due to smooth muscle constriction as well as inflammation and thickening within the small- and medium-sized bronchi raise frictional resistance and reduce airflow. “Scooping” of the flow-volume loop is caused by reduction of airflow, especially at lower lung volumes. Often, airflow obstruction can be reversed by inhalation of β2-adrenergic agonists acutely or by treatment with inhaled steroids chronically. TLC usually remains normal (although elevated TLC is sometimes seen in long-standing asthma), but FRC may be dynamically elevated. RV is often increased due to exaggerated airway closure at low lung volumes, and this elevation of RV reduces FVC. Because central airways are narrowed, Raw is usually elevated. Mild arterial hypoxemia is often present due to perfusion of relatively underventilated alveoli distal to obstructed airways (and is responsive to oxygen supplementation), but DLCO is normal or mildly elevated.
Airflow Obstruction Due to Decreased Elastic Recoil—Example: Severe Emphysema Loss of lung elastic recoil in severe emphysema results in pulmonary hyperinflation, of which elevated TLC is the hallmark. FRC is more severely elevated due both to loss of lung elastic recoil and to dynamic hyperinflation—the same phenomenon as autoPEEP, which is the positive end-expiratory alveolar pressure that occurs when a new breath is initiated before the lung volume is allowed to return to FRC. Residual volume is very severely elevated because of airway closure and because exhalation toward RV may take so long that RV cannot be reached before the patient must inhale again. Both FVC and FEV1 are markedly decreased, the former because of the severe elevation of RV and the latter because loss of lung elastic recoil reduces the pressure driving maximal expiratory flow and also reduces tethering open of small intrapulmonary airways. The flow-volume loop demonstrates marked scooping, with an initial transient spike of flow attributable largely to expulsion of air from collapsing central airways at the onset of forced exhalation. Otherwise, the central airways remain relatively unaffected, so Raw is normal in “pure” emphysema. Loss of alveolar surface and capillaries in the alveolar walls reduces DLCO; however, because poorly ventilated emphysematous acini are also poorly perfused (due to loss of their capillaries), arterial hypoxemia usually is not seen at rest until emphysema becomes very severe. However, during exercise, PaO2 may fall precipitously if extensive destruction of the pulmonary vasculature prevents a sufficient increase in cardiac output and mixed venous oxygen content falls substantially. Under these circumstances, any venous admixture through low /
units has a particularly marked effect in lowering mixed arterial oxygen tension.
FUNCTIONAL MEASUREMENTS
Measurement of Ventilatory Function • LUNG VOLUMES Figure 306e-2 demonstrates a spirometry tracing in which the volume of air entering or exiting the lung is plotted over time. In a slow vital capacity maneuver, the subject inhales from FRC, fully inflating the lungs to TLC, and then exhales slowly to RV; VC, the difference between TLC and RV, represents the maximal excursion of the respiratory system. Spirometry discloses relative volume changes during these maneuvers but cannot reveal the absolute volumes at which they occur. To determine absolute lung volumes, two approaches are commonly used: inert gas dilution and body plethysmography. In the former, a known amount of a nonabsorbable inert gas (usually helium or neon) is inhaled in a single large breath or is rebreathed from a closed circuit; the inert gas is diluted by the gas resident in the lung at the time of inhalation, and its final concentration reveals the volume of resident gas contributing to the dilution. A drawback of this method is that regions of the lung that ventilate poorly (e.g., due to airflow obstruction) may not receive much inspired inert gas and so do not contribute to its dilution. Therefore, inert gas dilution (especially in the single-breath method) often underestimates true lung volumes.
In the second approach, FRC is determined by measuring the compressibility of gas within the chest, which is proportional to the volume of gas being compressed. The patient sits in a body plethysmograph (a chamber usually made of transparent plastic to minimize claustrophobia) and, at the end of a normal tidal breath (i.e., when lung volume is at FRC), is instructed to pant against a closed shutter, thus periodically compressing air within the lung slightly. Pressure fluctuations at the mouth and volume fluctuations within the body box (equal but opposite to those in the chest) are determined, and from these measurements the thoracic gas volume is calculated by means of Boyle’s law. Once FRC is obtained, TLC and RV are calculated by adding the value for inspiratory capacity and subtracting the value for expiratory reserve volume, respectively (both values having been obtained during spirometry) (Fig. 306e-2). The most important determinants of healthy individuals’ lung volumes are height, age, and sex, but there is considerable additional normal variation beyond that accounted for by these parameters. In addition, race influences lung volumes; on average, TLC values are ~12% lower in African Americans and 6% lower in Asian Americans than in Caucasian Americans. In practice, a mean “normal” value is predicted by multivariate regression equations using height, age, and sex, and the patient’s value is divided by the predicted value (often with “race correction” applied) to determine “percent predicted.” For most measures of lung function, 85–115% of the predicted value can be normal; however, in health, the various lung volumes tend to scale together. For example, if one is “normal big” with a TLC 110% of the predicted value, then all other lung volumes and spirometry values will also approximate 110% of their respective predicted values. This pattern is particularly helpful in evaluating airflow, as discussed below.
AIR FLOW As noted above, spirometry plays a key role in lung volume determination. Even more often, spirometry is used to measure airflow, which reflects the dynamic properties of the lung. During an FVC maneuver, the patient inhales to TLC and then exhales rapidly and forcefully to RV; this method ensures that flow limitation has been achieved, so that the precise effort made has little influence on actual flow. The total amount of air exhaled is the FVC, and the amount of air exhaled in the first second is the FEV1; the FEV1 is a flow rate, revealing volume change per time. Like lung volumes, an individual’s maximal expiratory flows should be compared with predicted values based on height, age, and sex. While the FEV1/FVC ratio is typically reduced in airflow obstruction, this condition can also reduce FVC by raising RV, sometimes rendering the FEV1/FVC ratio “artifactually normal” with the erroneous implication that airflow obstruction is absent. To circumvent this problem, it is useful to compare FEV1 as a fraction of its predicted value with TLC as a fraction of its predicted value. In health, the results are usually similar. In contrast, even an FEV1 value that is 95% of its predicted value may actually be relatively low if TLC is 110% of its respective predictied value. In this case, airflow obstruction may be present, despite the “normal” value for FEV1.
The relationships among volume, flow, and time during spirometry are best displayed in two plots—the spirogram (volume vs. time) and the flow-volume loop (flow vs. volume) (Fig. 306e-4). In conditions that cause airflow obstruction, the site of obstruction is sometimes correlated with the shape of the flow-volume loop. In diseases that cause lower airway obstruction, such as asthma and emphysema, flows decrease more rapidly with declining lung volumes, leading to a characteristic scooping of the flow-volume loop. In contrast, fixed upper-airway obstruction typically leads to inspiratory and/or expiratory flow plateaus (Fig. 306e-4).
AIRWAYS RESISTANCE The total resistance of the pulmonary and upper airways is measured in the same body plethysmograph used to measure FRC. The patient is asked once again to pant, but this time against a closed and then opened shutter. Panting against the closed shutter reveals the thoracic gas volume as described above. When the shutter is opened, flow is directed to and from the body box, so that volume fluctuations in the box reveal the extent of thoracic gas compression, which in turn reveals the pressure fluctuations driving flow. Simultaneous measurement of flow allows the calculation of lung resistance (as flow divided by pressure). In health, Raw is very low (<2 cmH2O/L per second), and half of the detected resistance resides within the upper airway. In the lung, most resistance originates in the central airways. For this reason, airways resistance measurement tends to be insensitive to peripheral airflow obstruction.
RESPIRATORY MUSCLE STRENGTH To measure respiratory muscle strength, the patient is instructed to exhale or inhale with maximal effort against a closed shutter while pressure is monitored at the mouth. Pressures greater than ±60 cmH2O at FRC are considered adequate and make it unlikely that respiratory muscle weakness accounts for any other resting ventilatory dysfunction that is identified.
Measurement of Gas Exchange • DIFFUSING CAPACITY (DLCO) This test uses a small (and safe) amount of carbon monoxide (CO) to measure gas exchange across the alveolar membrane during a 10-sec breath hold. CO in exhaled breath is analyzed to determine the quantity of CO crossing the alveolar membrane and combining with hemoglobin in red blood cells. This “single-breath diffusing capacity” (DLCO) value increases with the surface area available for diffusion and the amount of hemoglobin within the capillaries, and it varies inversely with alveolar membrane thickness. Thus, DLCO decreases in diseases that thicken or destroy alveolar membranes (e.g., pulmonary fibrosis, emphysema), curtail the pulmonary vasculature (e.g., pulmonary hypertension), or reduce alveolar capillary hemoglobin (e.g., anemia). Single-breath diffusing capacity may be elevated in acute congestive heart failure, asthma, polycythemia, and pulmonary hemorrhage.
ARTERIAL BLOOD GASES The effectiveness of gas exchange can be assessed by measuring the partial pressures of oxygen and CO2 in a sample of blood obtained by arterial puncture. The oxygen content of blood (CaO2) depends upon arterial saturation (%O2Sat), which is set by PaO2, pH, and PaCO2 according to the oxyhemoglobin dissociation curve. CaO2 can also be measured by oximetry (see below):
CaO2 (mL/dL) = 1.39 (mL/dL) × [hemoglobin](g) × % O2 Sat
+ 0.003 (mL/dL/mmHg) × PaO2 (mmHg)
If hemoglobin saturation alone needs to be determined, this task can be accomplished noninvasively with pulse oxymetry.
ACKNOWLEDGMENT
The authors wish to acknowledge the contributions of Drs. Steven E. Weinberger and Irene M. Rosen to this chapter in previous editions as well as the helpful contributions of Drs. Mary Strek and Jeffrey Jacobson.
307 | Diagnostic Procedures in Respiratory Disease |
The diagnostic modalities available for assessing the patient with suspected or known respiratory system disease include imaging studies and techniques for acquiring biologic specimens, some of which involve direct visualization of part of the respiratory system. Methods to characterize the functional changes developing as a result of disease, including pulmonary function tests and measurements of gas exchange, are discussed in Chap. 306e.
IMAGING STUDIES
ROUTINE RADIOGRAPHY
Routine chest radiography, including both posteroanterior (PA) and lateral views, is an integral part of the diagnostic evaluation of diseases involving the pulmonary parenchyma, the pleura, and, to a lesser extent, the airways and the mediastinum (see Chaps. 305 and 308e). Lateral decubitus views are useful for determining whether pleural abnormalities represent freely flowing fluid, whereas apical lordotic views can visualize disease at the lung apices better than the standard PA view. Portable equipment is often used for acutely ill patients who cannot be transported to a radiology suite but are more difficult to interpret owing to several limitations: (1) the single anteroposterior (AP) projection obtained; (2) variability in over- and underexposure of film; (3) a shorter focal spot-film distance leading to lack of edge sharpness and loss of fine detail; and (4) magnification of the cardiac silhouette and other anterior structures by the AP projection. Common radiographic patterns and their clinical correlates are reviewed in Chap. 308e.
Advances in computer technology have allowed the development of digital or computed radiography, which has several benefits: (1) immediate availability of the images; (2) significant postprocessing analysis of images to improve diagnostic information; and (3) ability to store images electronically and to transfer them within or between health care systems.
ULTRASOUND
Diagnostic ultrasound (US) produces images using echoes or reflection of the US beam from interfaces between tissues with differing acoustic properties. US is nonionizing and safe to perform on pregnant patients and children. It can detect and localize pleural abnormalities and is a quick and effective way of guiding percutaneous needle biopsy of peripheral lung, pleural, or chest wall lesions. US is also helpful in identifying septations within loculated collections and can facilitate placement of a needle for sampling of pleural liquid (i.e., for thoracentesis), improving the yield and safety of the procedure. Bedside availability makes it valuable in the intensive care setting. Real-time imaging can be used to assess the movement of the diaphragm. Because US energy is rapidly dissipated in air, it is not useful for evaluation of the pulmonary parenchyma and cannot be used if there is any aerated lung between the US probe and the abnormality of interest.
Endobronchial US, in which the US probe is passed through a bronchoscope, is a valuable adjunct to bronchoscopy, allowing identification and localization of pathology adjacent to airway walls or within the mediastinum.
NUCLEAR MEDICINE TECHNIQUES
Nuclear imaging depends on the selective uptake of various compounds by organs of the body. In thoracic imaging, these compounds are concentrated by one of three mechanisms: blood pool or compartmentalization (e.g., within the heart), physiologic incorporation (e.g., bone or thyroid) and capillary blockage (e.g., lung scan). Radioactive isotopes can be administered by either the IV or inhaled routes or both. When injected intravenously, albumin macroaggregates labeled with technetium-99m (99mTc) become lodged in pulmonary capillaries; the distribution of the trapped radioisotope follows the distribution of blood flow. When inhaled, radiolabeled xenon gas can be used to demonstrate the distribution of ventilation. Using these techniques, ventilation-perfusion lung scanning was a commonly used technique for the evaluation of pulmonary embolism. Pulmonary thromboembolism produces one or more regions of ventilation-perfusion mismatch (i.e., regions in which there is a defect in perfusion that follows the distribution of a vessel and that is not accompanied by a corresponding defect in ventilation [Chap. 300]). However, with advances in computed tomography (CT) scanning, scintigraphic imaging has been largely replaced by CT angiography in patients with suspected pulmonary embolism.
Another common use of ventilation-perfusion scans is in patients with impaired lung function, who are being considered for lung resection. Many patients with bronchogenic carcinoma have coexisting chronic obstructive pulmonary disease (COPD), and the question arises as to whether or not a patient can tolerate lung resection. The distribution of the isotope(s) can be used to assess the regional distribution of blood flow and ventilation, allowing the physician to estimate the level of postoperative lung function.
COMPUTED TOMOGRAPHY
CT offers several advantages over routine chest radiography (Figs. 307-1A, B and 307-2A, B; see also Figs. 315-3, 315-4, and 322-4). First, the use of cross-sectional images allows distinction between densities that would be superimposed on plain radiographs. Second, CT is far better than routine radiographic studies at characterizing tissue density and providing accurate size assessment of lesions.
FIGURE 307-1 Chest x-ray (A) and computed tomography (CT) scan (B) from a patient with emphysema. The extent and distribution of emphysema are not well appreciated on plain film but clearly evident on the CT scan obtained.
FIGURE 307-2 Chest x-ray (A) and computed tomography (CT) scan (B) demonstrating a right lower-lobe mass. The mass is not well appreciated on the plain film because of the hilar structures and known calcified adenopathy. CT is superior to plain radiography for the detection of abnormal mediastinal densities and the distinction of masses from adjacent vascular structures.
CT is particularly valuable in assessing hilar and mediastinal disease (often poorly characterized by plain radiography), in identifying and characterizing disease adjacent to the chest wall or spine (including pleural disease), and in identifying areas of fat density or calcification in pulmonary nodules (Fig. 307-2). Its utility in the assessment of mediastinal disease has made CT an important tool in the staging of lung cancer (Chap. 107). With the additional use of contrast material, CT also makes it possible to distinguish vascular from nonvascular structures, which is particularly important in distinguishing lymph nodes and masses from vascular structures primarily in the mediastinum, and vascular disorders such as pulmonary embolism.
In high-resolution CT (HRCT), the thickness of individual cross-sectional images is ~1–2 mm, rather than the usual 7–10 mm in conventional CT. The visible detail on HRCT scans allows better recognition of subtle parenchymal and airway disease, thickened interlobular septa, ground-glass opacification, small nodules, and the abnormally thickened or dilated airways seen in bronchiectasis. Using HRCT, characteristic patterns are recognized for many interstitial lung diseases such as lymphangitic carcinoma, idiopathic pulmonary fibrosis, sarcoidosis, and eosinophilic granuloma. However, there is debate about the settings in which the presence of a characteristic pattern on HRCT eliminates the need for obtaining lung tissue to make a diagnosis.
Helical CT and Multidetector CT Helical scanning is currently the standard method for thoracic CT. Helical CT technology results in faster scans with improved contrast enhancement and thinner collimation. Images are obtained during a single breath-holding maneuver that allows less motion artifact and collection of continuous data over a larger volume of lung than is possible with conventional CT. Data from the imaging procedure can be reconstructed in coronal or sagittal planes (Fig. 307-3A), as well as the traditional cross-sectional (axial) view.
FIGURE 307-3 Spiral computed tomography (CT) with reconstruction of images in planes other than axial view. Spiral CT in a lung transplant patient with a dehiscence and subsequent aneurysm of the anastomosis. CT images were reconstructed in the sagittal view (A) and using digital subtraction to view images of the airways only (B), which demonstrate the exact location and extent of the abnormality.
Further refinements in detector technology have allowed production of scanners with additional detectors along the scanning axis (z-axis). These multidetector CT (MDCT) scanners can obtain multiple slices in a single rotation that are thinner and can be acquired in a shorter period of time. This results in enhanced resolution and increased image reconstruction ability. As the technology has progressed, higher numbers (currently up to 64) of detectors are used to produce clearer final images. MDCT allows for even shorter breath holds, which are beneficial for all patients but especially children, the elderly, and the critically ill. However, it should be noted that despite the advantages of MDCT, there is an increase in radiation dose compared to single-detector CT to consider.
In MDCT, the additional detectors along the z-axis result in improved use of the contrast bolus. This and the faster scanning times and increased resolution have all led to improved imaging of the pulmonary vasculature and the ability to detect segmental and subsegmental emboli. CT pulmonary angiography (CTPA) also allows simultaneous detection of parenchymal abnormalities that may be contributing to a patient’s clinical presentation. Secondary to these advantages and increasing availability, CTPA has rapidly become the test of choice for many clinicians in the evaluation of pulmonary embolism; compared with pulmonary angiography, it is considered equal in terms of accuracy and with less associated risks.
VIRTUAL BRONCHOSCOPY
The three-dimensional (3D) image of the thorax obtained by MDCT can be digitally stored, reanalyzed, and displayed as 3D reconstructions of the airways down to the sixth to seventh generation. Using these reconstructions, a “virtual” bronchoscopy can be performed (Fig. 307-4). Virtual bronchoscopy has been proposed as an adjunct to conventional bronchoscopy in several clinical situations: It can allow accurate assessment of the extent and length of an airway stenosis, including the airway distal to the narrowing; it can provide useful information about the relationship of the airway abnormality to adjacent mediastinal structures; and it allows preprocedure planning for therapeutic bronchoscopy to help ensure the appropriate equipment is available for the procedure.
FIGURE 307-4 Virtual bronchoscopic image of the trachea. The view projected is one that would be obtained from the trachea looking down to the carina. The left and right main stem airways are seen bifurcating from the carina.
Virtual bronchoscopy can be used to help target the area of peripheral lung for endobronchial lung volume reduction surgery that is being used in the management of pulmonary emphysema. The extent of emphysema in each segmental region together with other anatomic details may help in choosing the most appropriate subsegments. However, software packages for the generation of virtual bronchoscopic images are relatively early in development, and their utilization and potential impact on patient care are still unknown. Electromagnetic navigational bronchoscopy systems (EMN or ENB) using virtual bronchoscopy have been developed to allow accurate navigation to peripheral pulmonary target lesions, using technology similar to a car global positioning system (GPS) unit.
POSITRON EMISSION TOMOGRAPHIC SCANNING
Positron emission tomographic (PET) scanning is commonly used to identify malignant lesions in the lung, based on their increased uptake and metabolism of glucose. The technique involves injection of a radiolabeled glucose analogue, [18F]-fluoro-2-deoxyglucose (FDG), which is taken up by metabolically active malignant cells. However, FDG is trapped within the cells following phosphorylation, and the unstable [18F] decays by emission of positrons, which can be detected by a specialized PET camera or by a gamma camera that has been adapted for imaging of positron-emitting nuclides. This technique has been used in the evaluation of solitary pulmonary nodules and in staging lung cancer. Detection or exclusion of mediastinal lymph node involvement and identification of extrathoracic disease can be achieved. The limited anatomical definition of radionuclide imaging has been improved by the development of hybrid imaging that allows the superimposition of PET and CT images, a technique known as functional–anatomical mapping. Hybrid PET/CT scans provide images that help pinpoint the abnormal metabolic activity to anatomical structures seen on CT and provide more accurate diagnoses than the two scans performed separately. FDG-PET can differentiate benign from malignant lesions as small as 1 cm. However, false-negative findings can occur in lesions with low metabolic activity such as carcinoid tumors and bronchioloalveolar cell carcinomas, or in lesions <1 cm in which the required threshold of metabolically active malignant cells is not present for PET diagnosis. False-positive results can be seen due to FDG uptake in inflammatory conditions such as pneumonia and granulomatous diseases.
MAGNETIC RESONANCE IMAGING
The role of magnetic resonance imaging (MRI) in the evaluation of respiratory system disease is less well-defined than that of CT. Magnetic resonance (MR) provides poorer spatial resolution and less detail of the pulmonary parenchyma and, for these reasons, is currently not considered a substitute for CT in imaging the thorax. However, the use of hyperpolarized gas in conjunction with MR has led to the investigational use of MR for imaging the lungs, particularly in obstructive lung disease. In addition, imaging performed during an inhalation and exhalation can provide dynamic information on lung function. Of note, MR examinations are difficult to obtain among several subgroups of patients. Patients who cannot lie still or who cannot lie on their backs may have MRIs that are of poor quality; some tests require patients to hold their breaths for 15–25 seconds at a time in order to get good MRIs. MRI is generally avoided in unstable and/or ventilated patients and those with severe trauma because of the hazards of the MR environment and the difficulties in monitoring patients within the MR room. The presence of metallic foreign bodies, pacemakers, and intracranial aneurysm clips also preclude use of MRI.
An advantage of MR is the use of nonionizing electromagnetic radiation. Additionally, MR is well suited to distinguish vascular from nonvascular structures without the need for contrast. Blood vessels appear as hollow tubular structures because flowing blood does not produce a signal on MRI. Therefore, MR can be useful in demonstrating pulmonary emboli, defining aortic lesions such as aneurysms or dissection, or other vascular abnormalities (Fig. 307-5) if radiation and IV contrast medium cannot be used. Gadolinium can be used as an intravascular contrast agent for MR angiography (MRA); however, synchronization of data acquisition with the peak arterial bolus is one of the major challenges of MRA. The flow of contrast medium from the peripheral injection site to the vessel of interest is affected by a number of factors including heart rate, stroke volume, and the presence of proximal stenotic lesions.
FIGURE 307-5 Magnetic resonance angiography image of the vasculature of a patient after lung transplant. The image demonstrates the detailed view of the vasculature that can be obtained using digital subtraction techniques. Images from a patient after lung transplant show the venous and arterial anastomosis on the right; a slight narrowing is seen at the site of the anastomosis, which is considered within normal limits and not suggestive of obstruction.
PULMONARY ANGIOGRAPHY
The pulmonary arterial system can be visualized by pulmonary angiography, in which radiopaque contrast medium is injected through a catheter placed in the pulmonary artery. When performed in cases of pulmonary embolism, pulmonary angiography demonstrates the consequences of an intravascular thrombus—either a defect in the lumen of a vessel (a filling defect) or an abrupt termination (cutoff) of the vessel. Other, less common indications for pulmonary angiography include visualization of a suspected pulmonary arteriovenous malformation and assessment of pulmonary arterial invasion by a neoplasm. The risks associated with modern arteriography are small, generally of greatest concern in patients with severe pulmonary hypertension or chronic kidney disease. With advances in CT scanning, MDCT angiography (MDCTA) is replacing conventional angiography for the diagnosis of pulmonary embolism.
MEDICAL TECHNIQUES FOR OBTAINING BIOLOGIC SPECIMENS
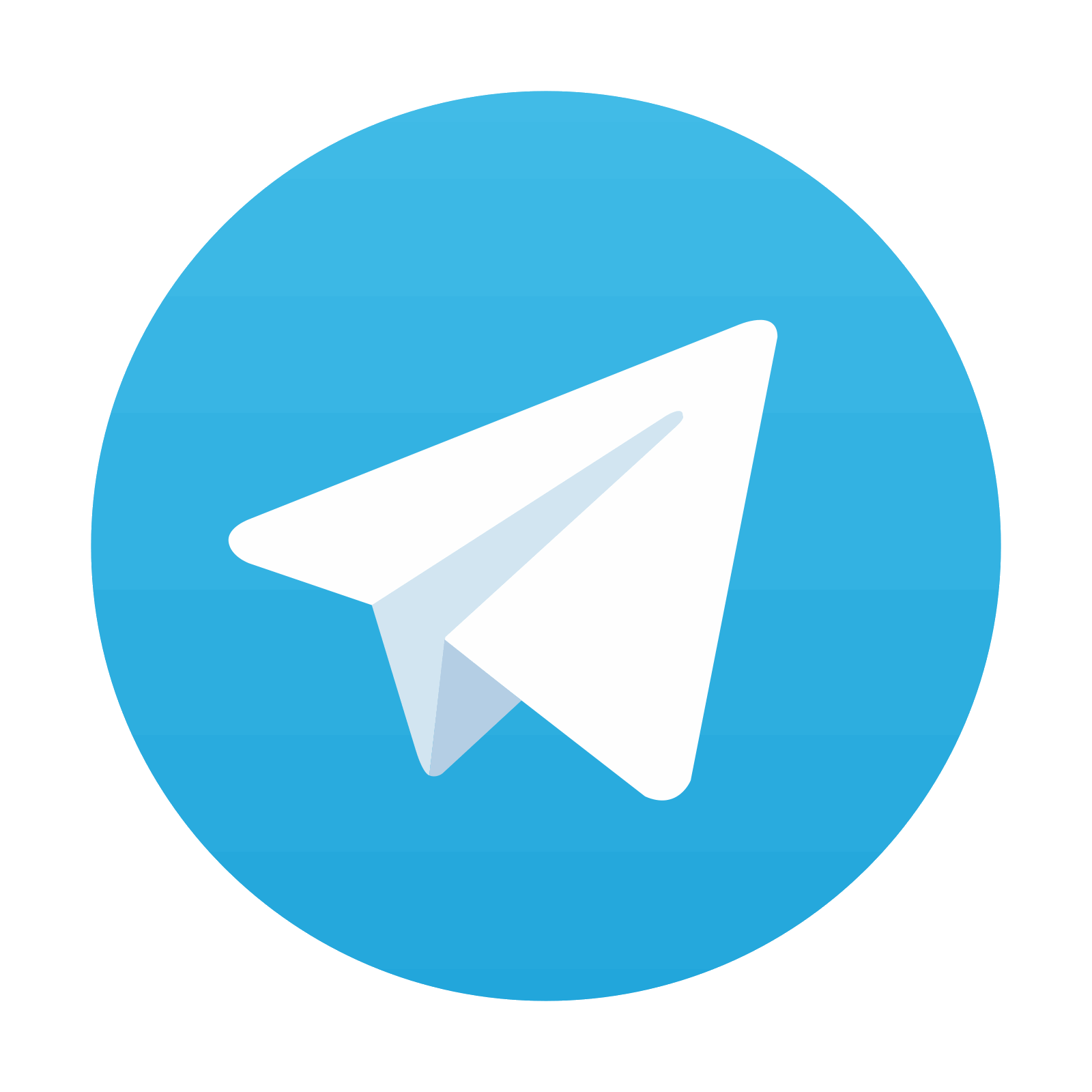
Stay updated, free articles. Join our Telegram channel
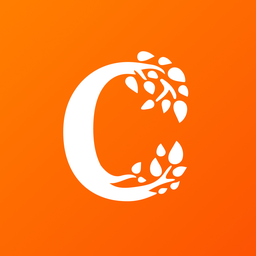
Full access? Get Clinical Tree
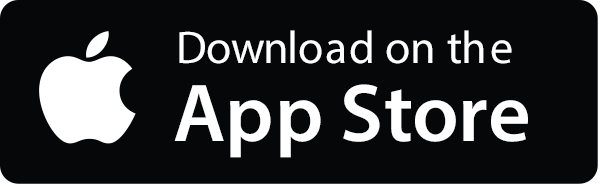
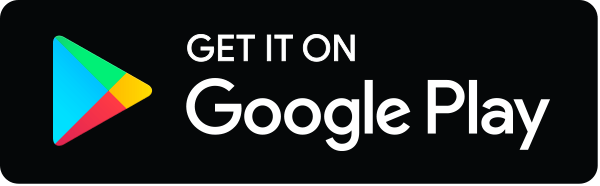