Chapter 1 Michael J. Caterina1,2,3,4,* 1 Department of Neurosurgery, Johns Hopkins School of Medicine, Baltimore, Maryland, USA The transient receptor potential (TRP) cation channel family consists of seven subfamilies that are widely expressed in mammalian tissues. By mediating flux of calcium, sodium, and other cations across cell membranes, in addition to nonionic signaling mechanisms, these channels contribute to many sensory and nonsensory processes throughout the body. Abnormalities in TRP channel function, whether a consequence of mutations in their sequence, alterations in their expression levels, or changes in their myriad regulators, have been associated with numerous disease states ranging from chronic pain to cardiovascular disease, skeletal abnormalities, and cancer. Such prevalent involvement in disease stems not only from the ubiquity of TRP channels but also from their complex pattern of polymodal gating. The connection between TRP channels and disease creates numerous opportunities for therapeutic intervention at these channels, whether through inhibition, activation, or co-opting of their ability to transport cations to alter the course of pathophysiological processes. The diverse repertoire of ion channels expressed in mammalian and nonmammalian species is encoded by a multitude of gene families. Among these, the transient receptor potential (TRP) ion channel family exhibits an especially prevalent and complex link with disease. Fittingly for the theme of this book, the TRP channel name emerged as a consequence of a disease state, although the victims of this disease were not human beings, but rather members of a line of visually impaired fruit flies [1]. Electroretinograms recorded from photoreceptors of these flies revealed that the electrical response to a light pulse (receptor potential), instead of remaining robust throughout a pulse of several seconds, decayed prematurely. Subsequent molecular and physiological studies revealed that the gene mutated in these so-called transient receptor potential (trp) flies encoded an ion channel subunit that, together with a homologous channel subunit, TRPL, forms the functional photoreceptor channel. This channel is not gated directly by light, but rather is activated by a G protein-coupled phospholipase C signaling pathway following the photoisomerization of the light receptor protein, rhodopsin. Following the identification of the Drosophila TRP channel, numerous homologous proteins were discovered, both in invertebrate species, such as fruit flies and nematodes, and in vertebrate species from fish to mammals [2]. Based on their domain structure and details of their sequences, members of the TRP channel family can be divided into seven subfamilies: TRPA (ankyrin, 1 human member), TRPC (canonical, 6 human members, plus 1 human pseudogene), TRPM (melastatin, 8 human members), TRPML (mucolipin, 3 human members), TRPN (NompC, no human members), TRPP (polycystin, 3 human members), and TRPV (vanilloid, 6 human members). There is also a distantly related family, TRPY, found in yeast. Functional TRP channels consist of homomeric or heteromeric tetramers of subunits from these subfamilies. The domain structure of an example TRP channel subunit, TRPV1, is shown in Figure 1.1a. A common structural feature of all TRP channel subunits is a core of six transmembrane domains (S1-S6), flanked by intracellular amino- and carboxyl-termini. Between S5 and S6 there is a complex pore-loop structure, which breaches the extracellular plane of the plasma membrane and forms the ion selectivity filter. This overall architecture resembles that of the voltage-gated and cyclic nucleotide-gated channel families. The TRPC, TRPM, TRPV, TRPA, and TRPN subfamilies, referred to as Group I TRP channels, resemble one another more closely than they do the TRPP or TRPML subfamilies, which are classified as Group II. Two features found among most Group I TRP channels include a TRP box homology element (absent in the TRPA subfamily), just distal to the sixth transmembrane domain, that participates in channel multimerization and modulation of gating, and a string of 4-16 sequential ankyrin repeat domains in the amino terminus (absent in the TRPM subfamily) that serves as a site of channel regulation (Figure 1.1a). Several TRPM subfamily members also contain kinase or nucleotide binding domains within their carboxyl termini and are therefore referred to as “chanzymes.” Ion flux through TRP channels occurs via a central pore lined by the pore loop domains of the four channel subunits (Figure 1.1b). All known TRP channels are selective for cations, although their degree of discrimination among cations can vary. For example, although some channels such as TRPV5 and TRPV6 are highly selective for Ca2 +, and TRPM4 and TRPM5 are relatively Ca2 + impermeant, most TRP channels are nonselective cation channels that can mediate flux of multiple monovalent and divalent cations [3]. Whereas most of these channels function at the plasma membrane, some are also found in organellar membranes. For example, TRPM2, TRPML, and TRPV2 channels can reside and function within the endolysosomal pathway [4]. A higher-resolution understanding of structural features of TRP channels has recently emerged with the solution of the atomic-level structure of one family member, TRPV1, by cryo-electron microscopy (Figure 1.1b and c) [5,6]. A few details and implications of this important advance will be described later in this chapter. TRP channels as a family are broadly expressed in mammalian tissues. In fact, every cell in the body likely expresses at least one family member, and often more. Moreover, these channels can be activated by a number of heterogeneous stimuli, including a plethora of endogenous and exogenous chemical ligands, physical stimuli such as temperature and mechanical force, free cytosolic Ca2 + ions, depletion of endoplasmic reticulum Ca2 + stores, and many others. It should therefore not be surprising that these channels have been linked to numerous physiological functions. The following examples provide a glimpse into the ubiquitous involvement of TRP channels in the fundamental processes of life. As will be emphasized later in this chapter, and throughout this book, the pervasiveness of TRP channels in normal mammalian biology sets the stage for them to serve as contributors to, modulators of, or even primary causes of numerous human diseases. Perhaps the best understood physiological functions of TRP channels are in the realm of sensory signal transduction. Just as the Drosophila TRP channel is a key effector in phototransduction, many other TRP channels serve either as primary transducers of environmental stimuli or as amplifiers or modulators of signals transduced by other receptors. The most extensively studied example from mammalian systems is TRPV1, a channel expressed at disproportionately high levels in a subpopulation of primary afferent nociceptors, sensory neurons that trigger the perception of pain [7]. TRPV1 was discovered on the basis of, and derives its name from, its ability to be gated by painful vanilloid compounds such as capsaicin (the main pungent ingredient in chili peppers) and resiniferatoxin (a highly potent irritant produced in the latex of Euphorbia plant species). Functional studies subsequently revealed that TRPV1 could alternatively be activated by other, nonvanilloid stimuli, most notably noxious heat (> 42 °C), protons (< pH 6), and various lipid metabolites. Activation of TRPV1 triggers calcium and sodium ion flux into nociceptor terminals, resulting in depolarization and consequent action potential firing. It also causes local neuronal release of bioactive peptides and consequent neurogenic inflammation. The necessity of TRPV1 for responsiveness to vanilloids and for normal heat-evoked pain has been demonstrated through the study of TRPV1 knockout mice, as well as the development and application of highly selective TRPV1 antagonists [7,8]. Subsequent to the discovery of TRPV1, a number of other TRP channels were identified as mediators of chemosensation, thermosensation, and perhaps most controversially, mechanosensation. Several examples include TRPA1, which is activated by pungent cysteine-reactive compounds such as mustard oil and acrolein, is a key mediator of itch sensation, and also appears to play roles in cold- and mechanically evoked pain [9]; TRPM8, which is activated by menthol and moderately cold temperatures and which is essential for mouse avoidance of such temperatures [10]; TRPC1, which has been implicated in transduction of low-intensity mechanical stimuli [11]; and TRPM3, another heat-gated channel that appears to contribute to heat-evoked pain [12]. In many cases, these channels appear to be functionally important not only at the peripheral terminals of sensory neurons, but also at their spinal and trigeminal central terminals, where they modulate the release of neurotransmitters onto second-order neurons [13,14]. Beyond somatosensory neurons, TRPM5 is a key participant in the detection of taste stimuli [15], whereas TRPC6 and TRPC7 appear to be involved in phototransduction by intrinsically photosensitive retinal ganglion cells [16]. Many TRP channels, including TRPC1, TRPC3, TRPC6, TRPM4, TRPV2, and TRPV4, are expressed in endothelial or muscle cells within the heart and blood vessels, where they regulate vascular tone and permeability, as well as cardiac contractility [17]. These functions are accomplished predominantly by increasing free cytoplasmic calcium levels. Many TRP channels are expressed intrinsically within the gastrointestinal tract [18]. For example, TRPV6 is a key effector of vitamin D-stimulated calcium absorption in the gut, whereas TRPV2 is expressed in enteric neurons, where it appears to regulate gastrointestinal motility. There is also abundant innervation of the gastrointestinal tract by extrinsic sensory afferents that express TRPV1, TRPA1, and other TRP channels. These neuronal channels regulate such processes as mucosal bloodflow and sensitivity to luminal distension. Within the exocrine portion of the gastrointestinal tract, TRPC1 and TRPV4 regulate saliva production and/or pancreatic acinar cell secretion, through the modulation of intracellular calcium levels and the regulation of aquaporin water channels. In the endocrine pancreas, TRPM5 and TRPM2, among others, contribute to the regulation of beta cell insulin release. TRP channels contribute to multiple processes within the kidneys and lower urinary tract [19–21]. These include regulation of renal glomerular filtration function by TRPC6, regulation of nephron osmoregulatory function by TRPV4, regulation of calcium and magnesium uptake by TRPV5 and TRPV6, and the contribution of TRPV1, TRPA1, and TRPV4 to the detection of stretch and irritation by bladder afferents and the urinary bladder epithelium. All the TRP channel subfamilies expressed in humans have been implicated in important functions within the central nervous system [22]. Examples include neurotransmitter release (TRPC3, TRPV1, TRPV2), neurogenesis (TRPC6), astrocyte calcium homeostasis (TRPA1), responses to oxidative stress (TRPM7, TRPM2), osmoregulation (TRPV1, TRPV4), respiratory control (TRPM4), and neuronal lysosomal function (TRPML). Among the immune cell processes demonstrated to involve TRP channels are regulation of T-cell membrane excitability (TRPM4) and macrophage and microglial phagocytosis, lysosomal acidification, and cytokine release (TRPM2, TRPM7, TRPV2) [23,24]. Other cell types that contribute to innate immune function, including skin keratinocytes and primary sensory afferents, also employ TRP channels to carry out these functions. For example, TRPA1 and TRPV4 were recently shown to mediate the release of immune modulatory molecules such as TSLP and endothelin-1 from keratinocytes [25,26], whereas TRPV1- and TRPA1-mediated neurogenic release of neuropeptides from sensory afferents can promote tissue swelling and regulate recruitment of immune cells [9,27]. TRP channels also perform important functions related to reproduction and embryonic development. For example, elimination of TRPM7 is lethal at very early embryonic stages [28]. TRPV3 controls differentiation of the skin and hair, at least in part by regulating keratinocyte production of EGF receptor ligands [29], whereas TRPV2, TRPV4, and TRPM7 all may be involved in the differentiation of human adipocytes [30]. Given the involvement of TRP channels in so many physiological processes, it should not be surprising that dysregulation of TRP channels has been linked to numerous pathophysiological conditions. In the ensuing chapters, the reader will encounter a host of situations in which an excess or shortage of TRP channel activity is a contributing factor to a human disease, or in which modulation of TRP channels provides a potential opportunity to circumvent a disease process. In some cases, the contribution of a particular TRP channel to a specific disease process is direct and paramount. As will be highlighted both in the chapter on hereditary TRP channelopathies (Chapter 2) and on TRP gene polymorphism (Chapter 4), as well as in chapters dedicated to diseases of specific organ systems, point mutations in a number of TRP channels are sufficient to produce clinical syndromes characterized by well-defined inheritance patterns [31,32]. Examples include mutations in TRPV4 leading to either sensorimotor axonopathies such as Type 2C Charcot-Marie-Tooth disease or heritable skeletal abnormalities such as brachyolmia, mutations in TRPA1 resulting in familial episodic pain syndrome, mutations in TRPC6 giving rise to focal segmental glomerulosclerosis, mutations in TRPV3 producing palmoplantar keratoderma and pruritus in Olmsted syndrome, mutations in TRPML3 causing the lysosomal storage disease type IV mucolipidosis, and mutations in TRPP1 and TRPP2 causing polycystic kidney disease. Although many of these are autosomal dominant conditions, this is not always so, and it is sometimes difficult to differentiate whether a given mutation produces disease through loss- or gain-of-function. There also exist situations in which the link between a given TRP channel and a given disease apparently arises from alterations in the expression of the channel or of factors that regulate the channel’s localization or activity. For example, following tissue inflammation or nerve injury, MAP kinase-dependent changes in the abundance of TRPV1 at the nociceptor terminal, protein kinase C and protein kinase A phosphorylation-mediated increase in TRPV1 sensitivity to its agonists or TRPV1 localization at the plasma membrane, and increases in the abundance of endogenous stimulators and potentiators of this channel, such as protons, endocannabinoids, and serotonin, all conspire to augment TRPV1 signaling [7,33]. A similarly complex regulatory pattern appears to exist for TRPA1 [9]. As a consequence of these events, TRPV1, TRPA1, and other TRP channels have emerged as key contributors to chemical, thermal, and mechanical hypersensitivity in animal models of nerve injury or inflammation. In humans, immunostaining studies have revealed upregulation of TRPV1 in peripheral nerves in a number of pain states [34]. Furthermore, a recent study showed that epigenetic changes in TRPA1 promoter methylation in white blood cells and gene expression in skin biopsies are predictive of human thermal pain sensitivity [35]. Finally, as discussed in two later chapters, the development of selective TRPV1 (Chapter 8) and TRPA1 (Chapter 9) antagonists has set the stage for the direct pharmacological assessment of the involvement of these channels in human disease [36]. As will be detailed throughout this book, many nonsensory pathophysiological conditions also appear to involve hyper- or hypoactivity of TRP channels. For example, abnormalities in TRP channel function in immune or inflammatory processes contribute to conditions such as asthma, dermatitis, gastrointestinal inflammation, and autoimmunity. TRP channels are also involved in cardiovascular diseases such as heart failure and hypertension and in both vascular and nonvascular diseases of the central nervous system. There is a also a growing body of evidence to support the intimate involvement of TRP channels, including TRPM8, TRPC6, TRPV2, TRPV1, and TRPM1, in cancer [37]. Finally, links have emerged between channels such as TRPM2, TRPV1, and TRPV4 and diseases involving tissue and organismal homeostasis, such as diabetes and obesity. Factors that contribute to the many diverse consequences of TRP channel dysfunction and likely explain the pervasive association of TRP channels with disease: (2) Importance of calcium signaling. The ability of many TRP channels to mediate calcium influx into cells makes them gatekeepers for one of the most potent of biological signals. Cytoplasmic calcium levels are normally tightly controlled and regulate many cellular processes, such as secretion, motility, action potential firing and propagation, neurotransmitter release, and gene expression. At the same time, high levels of calcium can be cytotoxic. This creates a situation in which either gain or loss of TRP channel function can profoundly influence cellular behavior and survival. (3) Diversity of signaling outputs. Calcium is not the only ion that can flow through TRP channels. As discussed earlier, many TRP channels are nonselective cation channels that can pass sodium, potassium, and magnesium ions, which in turn can influence cell behavior through the control of membrane polarization, cellular osmolarity, or more specific functions of the particular ions involved. In addition, some TRP channels, such as TRPV1, can be induced to mediate the influx of unusually large cations, such as potentially toxic aminoglycosides [38]. The repertoire of TRP channel signaling is expanded even further by the existence of catalytic domains on some subtypes, such as TRPM7, and the ability of TRP channels to functionally interact with other signaling proteins, such as Homer and STIM family members [39,40]. (4) Polymodal regulation and polygating. A remarkable feature of many TRP channels is that a given subtype can be alternatively regulated by multiple diverse stimuli that might be chemical, thermal, or mechanical in nature. Furthermore, multiple stimuli can converge on a given TRP channel to evoke additive, supra-additive, or antagonistic activities. Mutagenesis studies on many TRP channels, coupled with the recent solution of the TRPV1 atomic structure, have provided some insights into TRP channel polymodality. Examination of TRPV1 structures, solved in the absence vs. presence of exogenous activators [5,6], has revealed that multiple domains previously implicated in responsiveness to various agonists (a vanilloid binding pocket between transmembrane domains 3 and 4, a pore turret domain adjacent to the selectivity filter, the ankyrin repeat region that can bind and be modulated by intracellular ATP and calmodulin) are connected via conserved motifs (a linker prior to the first transmembrane domain, a helix between transmembrane domains 4 and 5, the helical TRP domain distal to transmembrane domain 6, a short pore helix adjacent to the selectivity filter) to not one, but two gates within the channel pore. The first gate is located toward the cytoplasmic end of the pore, at a site where the sixth transmembrane helices of each of the four subunits come in close proximity to restrict ion access to the pore. A similar (though not identical) gate has long been recognized in the voltage-gated channel family. The second, and somewhat surprising, gate in TRPV1 is located within the selectivity filter itself, which widens measurably with strong stimulation. The existence of this second gate explains why stimuli like protons and the tarantula-derived double-knot vanillotoxin activate the channel on binding to an adjacent pore turret region and may provide a hint at the structural basis for the observation that large cation permeability through TRPV1 is augmented by strong, persistent channel stimulation. Although the extreme carboxyl terminal domain of TRPV1 was not included in the structures solved to date, previous work suggests that phosphorylation of this domain, or its interaction with calmodulin, phosphoinositides, and other regulators [41], offers further opportunities for coupling with the inner and outer gates, most likely via the allosteric linker domains described earlier. Mutagenesis studies further suggest that similarly multiple, parallel but interconnected mechanisms for channel activation exist in other TRP channels [42]. Moreover, TRPV1 subunits, and thus probably subunits in other TRP channels, are clearly not autonomous of one another within the tetrameric holochannel. Rather, their regulatory and allosteric coupling domains are intricately interlaced with one another [5,6]. This remarkable interconnectedness among channel domains and subunits renders plausible a view of TRP channels as being exceptionally well poised to respond to diverse environmental signals at the slightest provocation and to function as coincidence detectors of multiple stimuli. This view is consistent with the observation that point mutations leading to constitutively active TRP channel function have been observed in many of the regulatory domains described earlier [32]. It also provides a potential explanation for how disease-related changes in the multitude of TRP channel regulators might manifest as disease. Given the numerous structural interactions required to maintain such allosteric complexity and thereby avoid unfettered calcium influx into the cell, it is amazing that, outside of a few well-conserved regions, the primary sequences of the many TRP channel family are actually quite divergent. For the same reason, it is a wonder that there are not more TRP channelopathies. Perhaps there are, but their incompatibility with life masks their true frequency. The prevalence of TRP channel involvement in human disease creates numerous opportunities, in principle, to develop therapeutic strategies based on these channels. A number of these strategies are described in detail in later chapters. In general, they fall into several categories outlined here. (1) TRP channel antagonists. In the case of diseases arising from TRP channel hyperactivity, specific small molecule antagonists offer the prospect of directly suppressing this activity. This strategy has been greatly facilitated by the fact that many TRP channels are “druggable,” in that they possess binding sites for small molecules such as the natural products (e.g., capsaicin, resiniferatoxin, cannabinoids, menthol) that have been used to characterize their functions. High throughput screens, often based on TRP channel-mediated influx of calcium in response to chemical activation, have enabled the isolation of numerous small molecules with high affinity and, in some cases, exquisite selectivity for particular TRP channel subtypes. As will be seen in later chapters, one potential complication associated with the therapeutic application of TRP channel antagonists, even if they are highly target specific, is interference with desirable physiological functions such as regulation of body temperature [8]. One approach to circumventing this potential hazard has been to develop peptides that prevent TRP channels from interacting with sensitizing binding partners. For example, A-kinase anchoring protein (AKAP) is an adaptor protein that facilitates the phosphorylation of TRPV1 by protein kinase C or protein kinase A. In animal models, peptides that mimic the TRPV1-recognition motif of AKAP have been shown to interfere with this interaction and thereby suppress hyperalgesia without impairing normal acute nociceptive function [43]. As an alternative strategy to avoid side effects, the complexity of TRP channel regulation described earlier also creates the potential opportunity to finely tune antagonists to interfere only with selected modalities of channel activation [8]. (2) TRP channel agonists. In certain disease states, it may be therapeutically desirable to stimulate, rather than inhibit, one or more TRP channels. Sometimes, the goal might be to exaggerate the normal physiological function of the channel for positive cellular benefit. For example, as described in Chapter 14, activators of TRPM8 might be useful to treat chronic pain because primary afferent inputs from cool-sensitive neurons expressing TRPM8 can apparently antagonize, at the spinal circuit level, inputs related to noxious heat [44]. In other cases, the goal is to achieve cytotoxicity through TRP channel-mediated calcium influx and thereby remove or neutralize a particular cell type. In the case of TRPV1, humans have been practicing this latter strategy for millennia through the regular consumption of spicy foods [45]. Whereas, acutely, exposure to capsaicin causes pain, prolonged exposure to this compound, or a more potent TRPV1 agonist, resiniferatoxin, desensitizes nociceptive neurons and eventually results in degeneration of nociceptor terminals (Chapter 6). Indeed, it was this observation that first led to the recognition that nociceptors constitute a neurochemically distinct subset of sensory neurons. As described in Chapter 7, this strategy has been explored not only for the treatment of pain, but also for the treatment of hyperactive bladder. Of note, this strategy is not necessarily aimed at specifically reducing the activity of the target TRP channel, but rather at more generally eliminating the function of the cell in which it is expressed. Another proposed use of cytotoxic hyperstimulation of TRP channels is to bring about apoptosis in tumor cells (Chapter 22). (3) TRP channel cargos. An especially clever TRP channel based therapeutic strategy exploits the unusual ability of some TRP channels, mentioned earlier, to mediate the influx of relatively large cations (mol. wt. > 600 Da). The best example of this strategy again involves TRPV1 and is based on the coadministration of capsaicin with a relatively large cationic molecule (QX314) that inhibits voltage-gated sodium channels through action at the intracellular end of the sodium channel pore [46]. Because capsaicin facilitates the entry of this cation through TRPV1, nociceptive neurons that express TRPV1 can be selectively loaded with relatively high concentrations of the sodium channel blocker, achieving therapeutically useful local concentrations without subjecting the recipient to potentially toxic systemic doses. (4) TRP channel gene therapy. An admittedly more ambitious approach that might prove useful to treat diseases resulting from TRP channel gain-of-function is genetic manipulation of TRP channel expression or sequence. For example, one could introduce an exogenous copy of a TRP channel cDNA, by viral transduction or other methods, to either rescue TRP channel hypofunction or to selectively drive ectopic expression of cytotoxic TRP channels in target cells to enhance agonist-stimulated elimination of those cells. RNA interference, antisense cDNAs, might be used to selectively reduce the expression of gain-of-function mutant TRP channels, whereas dominant negative TRP channels could be used to suppress the function of hyperfunctional or overly abundant endogenous TRP channels. Alternatively, gene editing using Cas9/CRISPR [47] or related tools might be used to repair mutated TRP channel genes or manipulate their promoters to modify channel expression. The diversity of TRP channels, their extraordinary physiological and pathophysiological importance, and the plethora of review articles and book chapters on these topics have inspired the invention of many variations of the TRP acronym. As will be evident throughout this book, TRP channels can fairly be viewed as both the problem and the potential solution in many human disease states. In keeping with the acronym tradition, it is therefore suggested that the reader view this incredible family of ion channels through the lens of their promise as Targets for the Resourceful Physician. M.J.C. is an inventor on a patent on the use of products related to TRPV1 and TRPV2, which is licensed through UCSF and Merck. This conflict is being managed by the Johns Hopkins Office on Policy Coordination.
An Introduction to Transient Receptor Potential Ion Channels and Their Roles in Disease
2 Department of Biological Chemistry, Johns Hopkins School of Medicine, Baltimore, Maryland, USA
3 Solomon H. Snyder Department of Neuroscience, Johns Hopkins School of Medicine, Baltimore, Maryland, USA
4 Neurosurgery Pain Research Institute, Johns Hopkins School of Medicine, Baltimore, Maryland, USA
* Corresponding author: caterina@jhmi.edu
Abstract
Discovery and General Properties of TRP Channels
TRP Channels in Normal Physiology
TRP Channels and Sensory Physiology
TRP Channels and Cardiovascular Function
TRP Channels and Gastrointestinal Function
TRP Channels and Urological Function
TRP Channels and the Brain
TRP Channels and Immune Function
TRP Channels and Development
TRP Channels and Disease
Therapeutic Strategies Based on TRP Channel Modulation
Acknowledgment
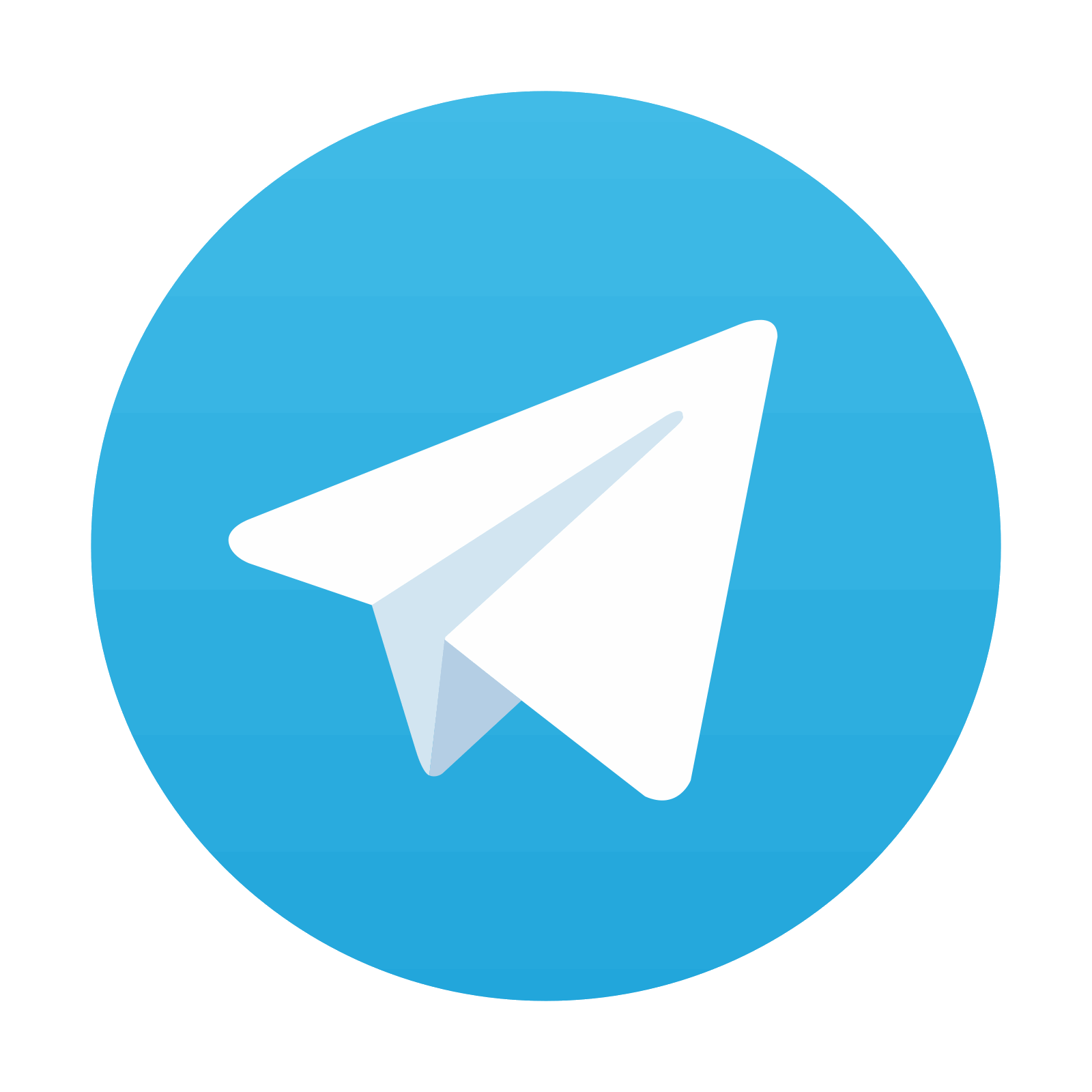
Stay updated, free articles. Join our Telegram channel
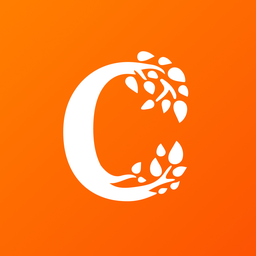
Full access? Get Clinical Tree
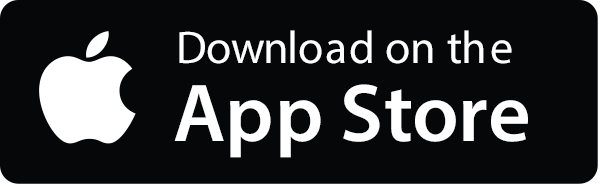
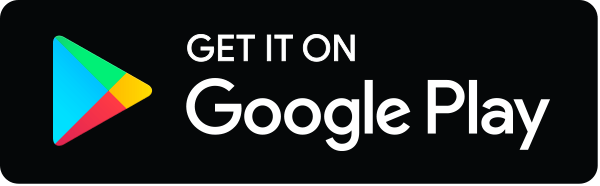