Adhesive pili of the chaperone-usher family
Vasilios Kalas, Ender Volkan and Scott J. Hultgren, Washington University School of Medicine, St. Louis, MO, USA
Introduction
Bacterial surface appendages are commonly divided into two categories: flagellar and non-flagellar. Flagella are long, propeller-like structures that provide motility to bacteria, distinct from non-flagellar structures known as pili or fimbriae, which are thinner, hair-like structures involved in adherence, biofilm formation, and in the case of type IV pili, twitching motility (see Chapter 13). The first functional observation of adhesive pili may have been in 1908, when Guyot recorded the ability of certain strains of bacteria to hemagglutinate red blood cells (Guyot, 1908). Today, hemagglutination is a common assay for detecting and measuring various types of adhesive pili (Korhonen et al., 1984; Hultgren et al., 1986; Krasan et al., 2000). The first images of non-flagellar surface structures were made possible in the late 1940s–early 1950s by the invention of the electron microscope (Houwink and van Iterson, 1950; Ottow, 1975). Duguid was an early pioneer in characterizing molecular features of pili and used the word ‘fimbriae’ in 1955, meaning threads or fibers in Latin, to describe the surface structures of Escherichia coli involved in erythrocyte agglutination (Duguid et al., 1955). He went on to serologically distinguish these fibers using different agglutination assays with erythrocytes from various species as well as with yeast cells (Gillies and Duguid, 1958; Duguid et al., 1966). The term ‘pili,’ Latin for hair, was later used by Brinton in 1959 to describe non-flagellar E. coli surface appendages (Brinton, 1959). Soon thereafter, he reported the first X-ray diffraction patterns of pili (Brinton, 1965). This consequently led Salit and Gotschlich to establish pilus purification procedures, perform early biochemical and functional characterizations, and describe the unique phenomenon of streaming birefringence exhibited by pili (Salit and Gotschlich, 1977). In the 1980s, work by Stanley Falkow and Staffan Normark unraveled a genetic understanding of pili (Hull et al., 1981; Normark et al., 1983), and in subsequent efforts, groups led by Stanley Falkow and Gordon Dougan were the first to clone pili gene clusters (Hull et al., 1981; Morrissey and Dougan, 1986). Meanwhile, afimbrial adhesins of E. coli were also discovered and cloned (Labigne-Roussel et al., 1985; Walz et al., 1985). These pioneering studies led to work focused on dissecting the biogenesis and structure of P pili of uropathogenic E. coli (UPEC), which provided a model and blueprint for the next three decades of work on these systems worldwide. The P pilus was discovered to be a multicomponent structure consisting of a stalk and an adhesive tip (Lindberg et al., 1987; Kuehn et al., 1992). At around the same time, PapD became the first pilus chaperone described in detail, a central factor in a molecular machine assembled by a process we would come to name the chaperone-usher (CU) pathway (Hultgren et al., 1989; Lindberg et al., 1989). The crystal structure of PapD described in 1989 was a landmark study that provided the first structural insights into CU pilus biogenesis (Holmgren et al., 1988; Holmgren and Branden, 1989). The surprising immunoglobulin (Ig)-like fold of PapD catapulted future structure–function analyses that led to the elucidation of fundamental principles of chaperone-assisted subunit folding and pilus biogenesis (Waksman and Hultgren, 2009). Thus, from the structural resolution of PapD and the discovery that it formed complexes with each of the pilus subunits, three decades of investigations were nucleated worldwide, which only recently culminated in the published crystal structure of an usher–chaperone–adhesin ternary complex (Phan et al., 2011). These historical findings served as the beginnings of a scientific era for understanding these virulence factors at the molecular level, which has now led to the development of therapeutics interfering with pilus assembly, pilus function, and hence, infection.
Pilus architecture
Pili of the CU system consist of multiple pilus subunits arranged into long, linear protein polymers. The morphology of CU pili varies across the six major clades – α, β, γ(1-4), κ, π, and σ – of the 189-membered CU pilus superfamily (as of 2007), ranging from thin, fibrillar structures to thick, helical rods topped by a fibrillar tip (Nuccio and Baumler, 2007). Paradigms for CU pilus architecture and assembly have been well established with the P pilus and type 1 pilus of UPEC, members of the π and γ1 clades, respectively. These two archetypal pili exhibit a bipartite organization, consisting of a long, helical rod connected to a thin tip fibrillum. The P pilus subunits PapG, PapF, PapE, PapK, PapA, and PapH arrange in order from fibrillar tip to rod base (Figure 12.1). PapG, the adhesin, lies at the distal end of the pilus. The tip adaptor PapF connects PapG to the main tip component PapE, which appears in 5–10 copies and has a width of ∼2 nm. The adaptor PapK anchors PapE to the main rod component PapA, which appears in >1000 copies and gives rise to a right-handed, helical structure that displays a 6.8 nm width, 2.5 nm pitch, and 3.3 subunits per turn (Kuehn et al., 1992; Jacob-Dubuisson et al., 1993; Striker et al., 1994). Finally, PapH attaches at the base of the rod and terminates pilus biogenesis (Baga et al., 1987; Verger et al., 2006). Type 1 pili adopt a similar, yet condensed architecture (Hahn et al., 2002). The fibrillum consists of single copies of both the adhesin FimH and the tip subunit FimG, while the adaptor FimF links the fibrillum to the major rod component FimA, which appears in ∼1000 copies (Figure 12.1). Preliminary studies suggest that FimI functions as the terminator subunit in type 1 pili (Valenski et al., 2003; Ignatov, 2009).
Chaperones
Structure and function
The chaperones of the CU pathway (∼25–30 kDa) are all highly homologous in sequence and structure (Hung et al., 1996). They are composed of two complete Ig-like domains (Figure 12.2A). Chaperones function to transiently bind their cognate subunits, shielding their interactive surfaces to facilitate their proper folding and stability in the periplasm (Kuehn et al., 1991).
In PapD, the chaperone from the P pilus system, a conserved salt bridge stabilizes the two Ig-like domains to adopt an overall boomerang-like shape. These domains orient to form a cleft that is directly involved in subunit binding. In the absence of subunits, chaperones like PapD and SfaE form dimers and Caf1M forms tetramers as a self-capping mechanism to prevent unfavorable interactions and proteolysis (Hung et al., 1999; Knight et al., 2002; Zavialov and Knight, 2007).
Interactive surfaces of the chaperone
Active site
Upon translocation of subunits across the SecYEG translocase to the periplasmic space, they are taken up by their cognate periplasmic chaperones, which use several interactive surfaces to provide stability to the subunits. The active site of the chaperone is comprised of residues R8 and K112, a conserved basic patch in the cleft formed between the two domains. Mutagenesis of these basic residues abolishes the ability of the chaperone to mediate pilus assembly (Slonim et al., 1992; Kuehn et al., 1993). Crystallography studies carried out on all of the P pili chaperone-subunit complexes (Sauer et al., 1999, 2002; Verger et al., 2006, 2007, 2008; Ford et al., 2012) further confirmed these interactions and the extended conformation by which the C-termini of subunits are anchored at the invariant R8 and K112 residues of the chaperone (Kuehn et al., 1993).
The G1 β-strand
The absence of the seventh β-strand in the subunit results in a deep groove on its surface that exposes its hydrophobic core. In a process termed donor strand complementation (DSC), the G1 strand of the chaperone is donated in trans in a non-canonical, parallel fashion to the exposed hydrophobic groove of the subunit to facilitate its proper folding (Sauer et al., 1999; Barnhart et al., 2000; Bann et al., 2004) (Figure 12.2B). The solvent-exposed set of alternating hydrophobic residues on the chaperone’s G1 strand directly interact with subunit pockets (Sauer et al., 1999). In the case of PapD, residues L107, I105, and L103 are respectively in register with the subunits’ hydrophobic P1–P3 pockets. Mutating residue 105 is particularly detrimental to pilus biogenesis (Hung et al., 1999). However, mutagenesis of residue L107 does not alter pilus assembly, which may be due to the plasticity of the P1 pocket (Ford et al., 2012). These residues are termed P1–P3 residues based on their corresponding acceptor sites, the subunit P1–P3 pockets (Figure 12.2D). Additionally, N101 forms hydrogen bonds above the shallow P4 subunit pocket. Thus, the interactions involved in DSC facilitate subunit folding and prevent subunit aggregation. The presence of unfolded subunits in the periplasmic space otherwise induces periplasmic stress responses targeting aggregated subunits for degradation by the DegP protease (Jones et al., 1997, 2002).
Once the subunit is folded, it remains bound to the chaperone until its incorporation into the pilus. The chaperone is exchanged for the N-terminal extension (Nte) of the incoming subunit via a ‘zip-in, zip-out’ mechanism termed donor strand exchange (DSE). DSE is initiated by the insertion of the Nte P5 residue into the open P5 pocket of the previously assembled subunit (Figure 12.2C–E). The chaperone G1 strand does not occupy the P5 pocket of subunits, which remains easily accessible in a chaperone–subunit complex to the P5 residue of the subunit Nte. Ultimately, insertion of Nte in an antiparallel fashion to the P2–P5 subunit pockets facilitates the removal of the chaperone’s parallel-oriented G1 strand. The DSE interaction is more energetically favorable than the DSC interaction, which allows DSE and the stable docking of subunits with each other to occur (Sauer et al., 2002; Remaut et al., 2006).
Usher-binding surface
PapD-like chaperones have a set of conserved, solvent-exposed, hydrophobic residues (termed Set B: L32, Q34, T53, P54, P55, V56, R68, I93) situated at the N-terminal domain (Figure 12.2A). X-ray crystallography studies demonstrated that the FimC chaperone of the type 1 pilus system interacts with the N-terminal domain of the usher via these Set B residues (Nishiyama et al., 2003; Eidam et al., 2008), demonstrating that the Set B patch is a surface that interacts with the usher. Accordingly, point mutations in these residues negatively impact pilus biogenesis (Hung et al., 1999). In addition, small molecules called pilicides, designed to block pilus biogenesis (Pinkner et al., 2006; Chorell et al., 2010), were shown to bind Set B residues and prevent the targeting of chaperone–subunit complexes to the usher (Pinkner et al., 2006). These results implicate PapD’s conserved hydrophobic patch Set B as the usher-targeting site. Other chaperone residues that may be involved in interactions with other domains of the usher are subjects of investigation.
Conserved cleft residues and the DRES motif
Another set of highly conserved residues (Set C: L78, P79, D81, R82, E83, S84) is located at the elbow region of PapD, which is not known to interact with any protein partners. The stability of the chaperone depends on the formation of a buried salt bridge composed of D196, E83, and R116, which lies at the interdomain region (Hung et al., 1999). E83 is part of the aspartate, arginine, glutamate, and serine (DRES) motif, which is highly conserved in the chaperone superfamily (Hung et al., 1996). Situated at the end of the E1–F1 loop, part of the DRES motif packs against the hinge region connecting the two domains (Holmgren and Branden, 1989; Hung et al., 1996), where the aspartate and arginine side chains from the DRES motif point out into solution, seemingly suitable for protein–protein interactions (Figure 12.2A). It is plausible that interactions with other proteins (subunits or usher domains) could cause conformational changes that would be transmitted to the interdomain region, possibly disrupting the salt bridge and thus facilitating a reorientation of the domains and causing allosteric conformational changes in the chaperone that play a role in the assembly of the CU pilus.
Subunits
Pilins
There are several major functional types of pilin that may comprise a CU pilus, including adhesins, adaptors, tip fibrillar subunits, pilus rod subunits, and terminators (Figure 12.1). Pilins, or pilus subunits, share an outstanding degree of sequence and structural homology with one another, necessary for preserving common mechanisms of subunit–subunit, chaperone–subunit, and usher–chaperone–subunit interactions needed for maturation of a functional pilus. As described, the incomplete nature of the pilin fold insures either chaperone binding (by DSC) or Nte binding (by DSE) to pilus subunits for their stability, proper folding, and ultimate incorporation into the growing pilus. Thus, the Nte, chaperone G1 strand, and the incomplete Ig-like fold of pilin subunits, particularly the P1–P5 pockets, serve as common recognition motifs in the CU pathway for the use of pilins as building blocks in the construction of a pilus.
However, structural distinctions across homologous pilins permit their unique positions and functions along the pilus chain. For example, differences in Nte sequence and hydrophobic pocket characteristics dictate subunit ordering. DSE reactions performed by incubating all combinations of Nte peptides (based on the five Nte-containing Pap subunits) with all chaperone–subunit complexes showed a range of reactivities (Rose et al., 2008; Verger et al., 2008). Reactions that occurred most rapidly were consistently those between cognate groove–Nte partners. In addition to chaperone–subunit and subunit–subunit interactions, subunits also contain specific surfaces that drive chaperone–subunit–usher interactions. Differences in subunit structure and residue side chains dictate selective trafficking to certain periplasmic domains of the usher (see Ushers: Domain function and selectivity). Thus, adhesin and terminator subunits, which occupy opposite ends of the pilus, adopt structures much more varied from their pilin counterparts required for their essential functions in host adhesion and pilus assembly termination, respectively.
Adhesin
A mature CU pilus typically contains an adhesin at its distal end. Adhesins contain two distinctive domains: a lectin domain, which functions to bind specific receptors with stereochemical specificity and contribute to host and tissue tropisms, and an Ig-like pilin domain, which links the receptor-binding lectin domain to the fibrillar tip (Choudhury et al., 1999; Sauer et al., 1999). FimH and PapG, adhesins of the prototypical type 1 and P pilus systems, respectively, mediate host–pathogen interactions important in cystitis (Wu et al., 1996; Mulvey et al., 1998; Martinez et al., 2000; Bahrani-Mougeot et al., 2002) and pyelonephritis (Roberts et al., 1994). FimH mediates binding to α-D-mannose-containing receptors whereas PapG binds galabiose, a receptor that is present in the globo series glycolipids found in the kidney. Both lectin domains contain a β-barrel jelly-roll fold that is common to CU adhesin structures, but they contain different receptor-binding pockets. FimH contains a deep, negatively charged pocket at the tip of the lectin domain (Hung et al., 2002). In contrast, PapGII (class II of three PapG classes, each of which recognize different globo series of glycolipids) binds galabiose-containing receptors with a shallow binding pocket formed by three beta strands and a loop at the side of the lectin domain (Dodson et al., 2001). CU adhesins F17-G of ETEC (Buts et al., 2003) and GafD of UPEC (Merckel et al., 2003) bind N-acetyl-D-glucosamine residues of proteins at a shallow binding site on the side of the lectin domain at a location unrelated to the binding pocket of PapG (Figure 12.3). With the function of adhesion segregated to a distinct protein domain at the pilus tip, various bacterial strains can alter the structure and receptor specificity of the adhesin, thus allowing flexibility and selective advantage in establishing tropism.
Numerous studies suggest that the lectin domain of FimH does not operate independently of the pilin domain but instead interacts intimately with it to influence receptor binding. A crystal structure of the type 1 pilus tip fibrillum (Le Trong et al., 2010) indicates a markedly different conformation in FimH, compared to the conformation observed in FimH in the FimC–FimH complex (Choudhury et al., 1999) or in the mannose-bound FimC–FimH structure (Hung et al., 2002). In the tip structure, the pilin domain interacts with a compacted lectin domain in a stable, non-covalent manner, loosening the mannose-binding pocket at the distal end of the lectin domain and thereby inducing an inactive low-affinity state of the adhesin via a ‘page-turning’ allosteric mechanism (Le Trong et al., 2010). In contrast, FimH, when in complex with FimC and α-D-mannose, adopts an elongated, high-affinity state (Hung et al., 2002), which was later shown to be induced by application of shear force, presumably by destabilization of interdomain contacts. Indeed, cross-linking mutants along with positively selected residues in the pilin domain suggest that this conformational equilibrium is important for mannose binding and infectivity in murine models of cystitis (Chen et al., 2009; Le Trong et al., 2010). Shear force does indeed enhance bacterial adhesion to target cells (Thomas et al., 2002), but whether this mechanism of tensile stress-induced binding enhancement applies to other pili systems or has a definitive role in pathogenesis remains to be seen.
Terminator
Studies in the Pap system suggest that pilus biogenesis ends with the incorporation of the PapH terminator at the base of the pilus rod. Deletions or mutations of PapH result in the formation of long pili that are shed from the extracellular surface (Baga et al., 1987). Overexpression of PapH results in short pili. Further, the structure of PapD–PapH indicates that PapH has an occluded P5 pocket, which prevents the initiation of DSE with further subunits, thereby terminating pilus biogenesis (Verger et al., 2006). Analogously, FimI may function as the terminator in type 1 pili (Valenski et al., 2003; Ignatov, 2009). Further studies will elucidate whether incorporation of a P5 pocket-lacking pilin is utilized by other CU pili for termination.
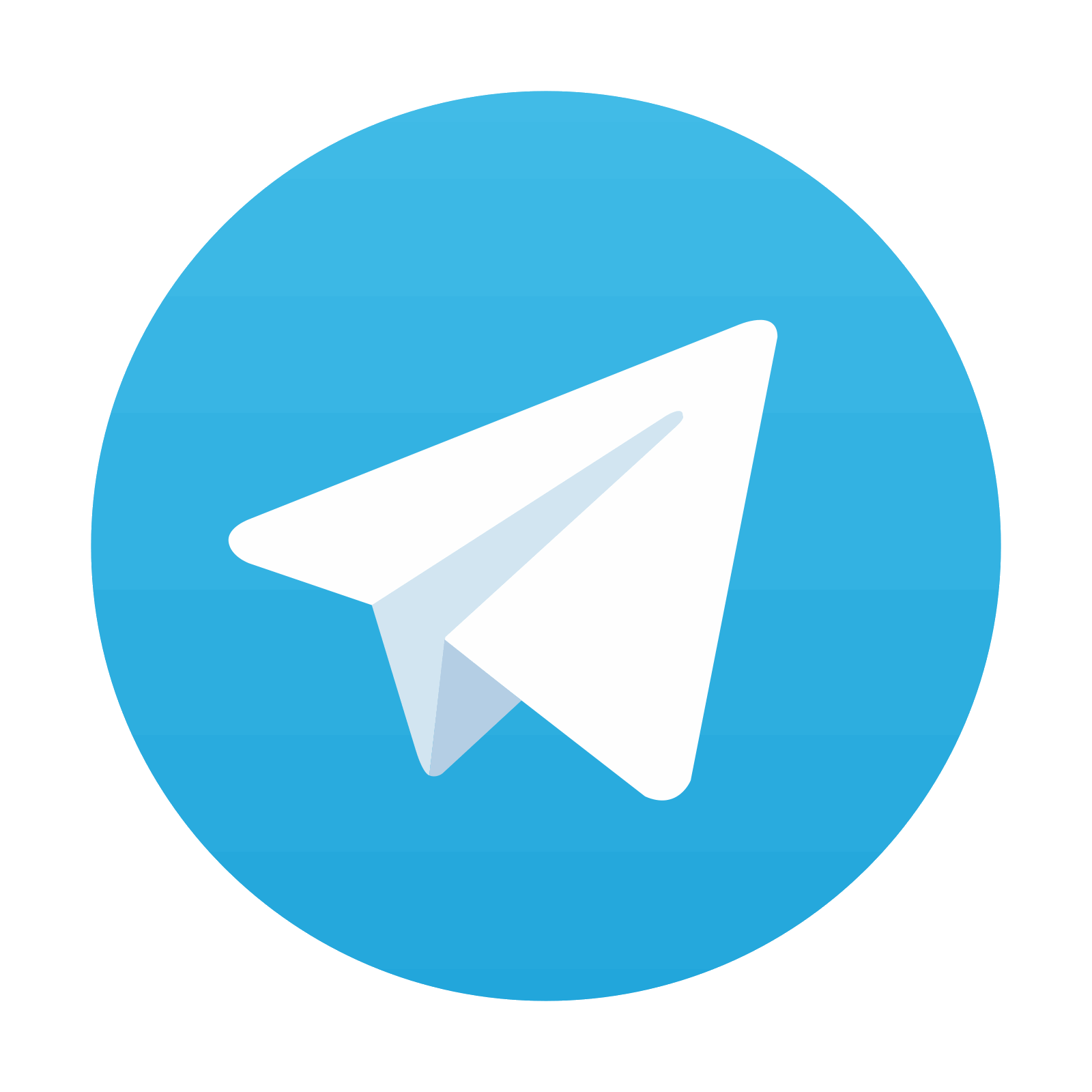
Stay updated, free articles. Join our Telegram channel
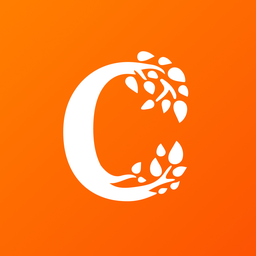
Full access? Get Clinical Tree
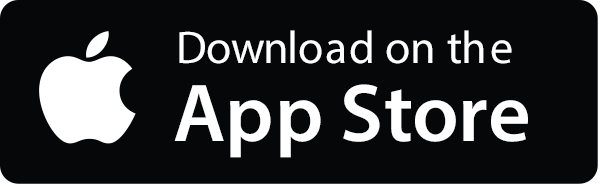
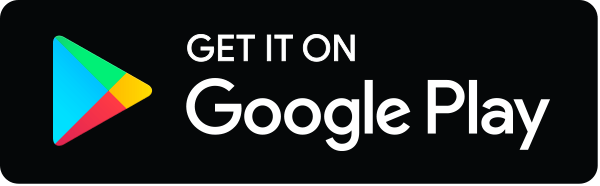