DIAGNOSIS & MANAGEMENT OF ALTERATIONS IN CONSCIOUSNESS
Alterations of consciousness and the related conditions of delirium, acute confusional state, and acute encephalopathy are among the most common mental disorders encountered in either surgical or medical patients. The prevalence of altered mental status in hospitalized patients is high, with reported rates up to 50%. These conditions are associated with increased mortality rates ranging from 10% to 65%, and excess annual health care expenditures in the billions. Given the high incidence of altered mental states, at least a basic understanding of the pathophysiology, diagnosis, and management of common etiologies is warranted for all medical practitioners.
Consciousness is generally defined as the subjective experience of the environment and the self. It is comprised of two components: arousal, which is the state of wakefulness, and awareness, which is the state of phenomenal perception. This distinction is useful, since the two processes are dissociable. For example, a vegetative state is characterized by a patient that is awake (ie, the cortex is aroused), but not necessarily aware.
Arousal is generated by activity of the ascending reticular activating system, which is composed of neurons within the central mesencephalic brainstem, the lateral hypothalamus, and portions of the thalamus. Widespread projections of these nuclei synapse on neurons in the cerebral cortex and generate an arousal response. Arousal responses define the level of consciousness (eg, being awake vs. asleep vs. comatose). Awareness is thought to be generated through networks involving the thalamus and association cortices of the frontal, parietal, temporal, and occipital lobes. Processes related to awareness define the content of consciousness (eg, seeing a blue circle vs. a red triangle).
Many terms are used to describe the levels of consciousness ranging from alert to comatose. The alert patient is awake and immediately responsive to all stimuli. Stupor is a condition in which the patient is less alert but still responds with stimulation. An obtunded patient appears to be asleep much of the time but still responds to noxious stimuli. A vegetative state is a state of arousal without awareness in which the patient may open his or her eyes, track objects, chew, and swallow, but not respond to auditory stimuli or appear to sense pain (although pain processing is now known to occur in the vegetative state). The comatose patient appears asleep and does not respond to stimuli. Often, terms used to describe states of consciousness lack consistent definitions, and a clear description of a patient’s state of arousal and awareness results in more precise communication.
In general, altered states of consciousness can arise from physiologic, pharmacologic, and pathologic causes. Before addressing pathologic causes (which can have structural or nonstructural etiologies), physiologic perturbations such as hypoglycemia, hypoxia, hypercarbia, hyponatremia, and hypothermia should be addressed. Pharmacologic etiologies, such as acute intoxication, overdose, and residual anesthesia from surgery, should also be considered and reversed when possible.
The reticular activating system is excited by a wide variety of stimuli, particularly somatosensory stimuli. Given that its nuclei are highly concentrated in the midbrain, it can be damaged by central midbrain lesions, which can result in the loss of arousal and coma.
Less severe dysfunction of the reticular activating system results in an acute confusional state. The cardinal signs of an acute confusional state are somnolence, inattention, and disorientation. Additionally, perceptions may be distorted, leading to hallucinations, and the patient may be unable to organize and interpret a complex stimuli. Disordered perception results in dysfunctional learning, memory, and problem solving. Thought processes can be disorganized and tangential, and the confused patient may develop delusions. In some cases, the acute confusional state presents as delirium, which can, in its hyperactive form, be characterized by heightened arousal, disordered perception, agitation, delusions, hallucinations, and autonomic hyperactivity (diaphoresis, tachycardia, hypertension, and mydriasis). However, it is important to note that hypoactive delirium is the most common type to be manifested in the postoperative setting.
Neurons in the pons, midbrain, and hypothalamus are necessary for regulation of sleep-wake cycles. Therefore, lesions involving the pons may preserve consciousness but disturb sleep. This is in contrast to the typical vegetative state that results from diffuse destruction or injury of the bilateral cerebral cortices, secondary to global cerebral ischemia or anoxia with preservation of the reticular activating system and brainstem sleep centers. This leaves the patient with preserved sleep-wake cycles without the ability to interact with the environment.
Acute confusional states and coma may result from structural or metabolic causes. Structural lesions of the cerebral hemispheres such as hemorrhage (intracerebral, subdural, or epidural), large areas of ischemic infarction, abscesses, or neoplasms can expand over minutes or a few hours and result in significant elevation of the intracranial pressure (ICP).
Several nonstructural disorders that diffusely disturb brain function can produce a confusional state or, if severe, coma (Table 36–1). Acute toxic-metabolic encephalopathy (TME), which encompasses delirium and the acute confusional state, is an acute condition of global cerebral dysfunction in the absence of primary structural brain disease. Typically, TME is usually a consequence of systemic disease or secondary to drugs and metabolic toxins that are reversible, thus making prompt recognition and treatment critical.
Physiologic | Pathologic | Pharmacologic |
---|---|---|
Global cerebral ischemia Hepatic encephalopathy Hypoxia/hypercarbia Hyperosmolar state Hypotension Hypo/hypercalcemia Hypo/hyperthermia Hypo/hyperglycemia Hypo/hypernatremia Hypothyroidism Thyrotoxicosis Uremia | Trauma-associated subdural or epidural hematoma Tumor Infection—meningitis or encephalitis Vascular (stroke) Ischemic stroke (ie, basilar artery thrombosis) Subarachnoid hemorrhage Intracerebral hemorrhage Seizures Subclinical status epilepticus Prolonged post-ictal state | Sedatives Narcotics Alcohol/illicit substances Poisoning |
Additionally, TME is common among critically ill patients and is probably underdiagnosed, especially when it occurs in patients who require mechanical ventilation. Risk factors for the development of TME include admission to an ICU, advanced age, preexisting primary neurodegenerative disease (dementia), nutritional deficiency, infection, temperature dysregulation, and failure of multiple organ systems.
The skull encloses three major components: brain parenchyma, cerebrospinal fluid (CSF), and blood. The volumetric sum of these components is maintained at a constant. Given the nondistensible cranial vault and these three noncompressible substances, an increase in one component must be offset by a decrease in another or pressure will increase. This offset is normally accomplished by displacement of CSF into the contiguous subarachnoid space of the spinal cord, termed “spatial compensation.” This delicate balance, however, has physiologic limits. Once these compensatory limits are met, an increase in the volume of any component will lead to an exponential increase in ICP as illustrated by the intracranial elastance curve (Figure 36–1).
Normal ICP in adults is less than 10-15 mm Hg. The presence of tumor or blood, the impedance of CSF drainage (hydrocephalus), cerebral edema, and increased cerebral blood flow (hyperemia as a response to head injury or hypoventilation causing hypercarbia and vasodilation) may raise the ICP to a dangerous level. The increased ICP may lead to reduced cerebral perfusion and ischemia and a mass effect progressing to shifting of the brain parenchyma and devastating herniation.
Increased ICP is often accompanied by altered mental status, headache, vomiting (without nausea), and papilledema on fundoscopic examination. Cushings triad may be seen in patients with severely increased ICP and consists of hypertension, bradycardia, and respiratory irregularity. Obtundation, focal neurologic findings including unilateral pupillary dilation from pressure or traction exerted on the third cranial nerve (ie, the “blown” pupil), and hemodynamic instability are late findings and indicate pending uncal herniation.
Cerebral herniation occurs when increased ICP results in a portion of the brain shifting from one intracranial compartment to another. These shifts may lead to vascular compromise and infarction of portions of the brain, disruption of white matter pathways, and direct pressure on structures such as cranial nerve III.
Occurs in patients with a frontal lobe mass as the cingulate gyrus herniates beneath the falx. Symptoms are often related to the mass or increased ICP.
Occurs in patients with an expanding lateral mass. Symptoms include contralateral hemiparesis, diminished consciousness, and an ipsilateral cranial nerve III palsy. Compression of pupillary fibers also causes a dilation of the pupil and as lateral displacement of the midbrain continues there is ipsilateral hemiplegia. Late in the process, the uncus and hippocampus may herniate transtentorally.
Posterior fossa masses cause symptoms by compression of the brainstem and obstructing the flow of CSF (hydrocephalus). As pressure increases, the cerebellar tonsils may be pushed into or through the foramen magnum. As the medulla is compressed, apnea results from dysfunction of the medullary respiratory center.
Posterior fossa masses may cause obstructive hydrocephalus. If these patients undergo ventriculostomy, there is a possibility of upward herniation of posterior fossa contents into the region of the thalamus and hypothalamus.
The diagnosis of an altered state of consciousness is provided by general physical examination, neuroradiological imaging, drug screens, and certain laboratory studies.
The neurologic exam includes assessment of level of consciousness, brainstem reflexes, and motor activity. Rapid, standardized assessment of the level of consciousness is useful for clinical decision-making and communication. The level of alertness reflects the severity of the underlying condition; severely affected patients are comatose. Commonly, the Glasgow Coma Scale (GCS) (Table 36–2) is used to assess level of consciousness in patients with head injury, but is also widely used as assessment of level consciousness regardless of etiology. A patient with a GCS score 8 or less is generally considered to be comatose. The cardinal feature of confusion and delirium is impaired attention. Simple bedside tasks such as serial subtraction or naming the months of the year in reverse can test attention. Marked fluctuations in mental status over time are characteristic. Other common findings include a disturbed sleep-wake cycle, decreased alertness, hypervigilance, hallucinations, sensory misperceptions, impaired memory, and disorientation. The thought process is often disorganized, manifested by confused or rambling conversation.
Eye Opening: | |
Spontaneous | 4 |
To voice | 3 |
To pain | 2 |
None | 1 |
Verbal Response: | |
Oriented | 5 |
Confused, disoriented | 4 |
Inappropriate words | 3 |
Incomprehensible sounds | 2 |
None | 1 |
Best Motor Response: | |
Obeys | 6 |
Localizes | 5 |
Withdraws (flexion) | 4 |
Abnormal flexion posturing | 3 |
Extension posturing | 2 |
None | 1 |
The cranial nerves and their corresponding brainstem reflexes, including pupillary response to light, corneal reflexes, oculocephalic reflex (doll’s eyes), vestibulo-ocular reflex (cold calorics), breathing patterns, cough, and gag reflexes are further used to describe accurately the neurologic status and localize the level of brainstem dysfunction. Generally, the brainstem reflexes are only affected in severe TME. Abnormal patterns of respiration, particularly Cheyne–Stokes respiration, may also occur with significant brainstem dysfunction.
Motor responses may be delineated as spontaneous or induced by noxious stimuli, purposeful or nonpurposeful, unilateral or bilateral, and upper or lower extremity. The patient may display withdrawal from a stimulus, abnormal flexion (decorticate posturing), abnormal extension (decerebrate posturing), or absence of motor activity. A variety of motor abnormalities are also associated with TME: tremor, asterixis, multifocal myoclonus (sudden, nonrhythmic gross muscle twitching), paratonia (increased tone with variable resistance), diffuse brisk deep tendon reflexes, or bilateral extensor plantar responses.
The laboratory investigation of decreased level of consciousness includes a complete blood count, coagulation studies, electrolyte panel, and examination of calcium, magnesium, phosphate, glucose, blood urea nitrogen, creatinine, bilirubin, liver enzymes, ammonia, serum osmolality, and arterial blood gases. Toxicology screening should be performed for suspected intoxications. Blood and CSF cultures should be obtained if infection appears present. Further analysis of CSF with cell counts, protein, glucose, and organism-specific studies such as herpes simplex virus PCR and fungal serology can reveal meningitis, neoplastic cells, or subarachnoid hemorrhage. Thyroid function tests and vitamin B12 and serum cortisol concentrations should be assessed if endocrinopathy is considered.
Emergent computed tomography (CT) imaging to assess for structural lesions (intracerebral hemorrhage, large territory ischemic stroke, hydrocephalus, neoplasm, and diffuse cerebral edema) is strongly recommended for patients presenting with focal neurological deficits or severe decline in the level of consciousness. Acute ischemic stroke within the first 3-4 hours may be clinically occult on CT imaging. Magnetic resonance imaging (MRI) of the head is generally reserved to further assess for acute ischemic stroke as well as further characterization of neoplastic lesions. MRI should be not relied upon for an emergent diagnosis given the duration of the study.
The electroencephalogram (EEG) can both confirm global cerebral dysfunction and exclude subclinical status epilepticus with greater sensitivity than clinical examination alone. After structural lesions have been excluded, EEG should be performed in most patients with altered consciousness at some point during the course of their care. The degree of diffuse slowing of the normal background plus abnormal mixed rhythms in the EEG correlates with the severity of TME. Slowing can be categorized as follows: mild, with a reduction in the normal alpha frequencies (8-13 Hz); moderate, with theta frequencies (4-8 Hz); severe, delta frequencies (less than 4 Hz).
Quantification of ICP is critical for management of patients with large intracerebral lesions resulting in mass effect and shift of midline structures, diffuse cerebral edema, or hydrocephalus. ICP cannot accurately be estimated based on clinical findings or imaging. There are several methods of direct ICP measurement, but the two most commonly employed in clinical practice are ventricular catheters and intraparenchymal microtransducer systems. Other methods such as subarachnoid and epidural devices have much lower accuracy.
The gold standard of ICP monitoring is via an intraventricular catheter connected to a standard pressure transducer. These catheters are usually placed into the lateral ventricle by a small frontal burr hole. The advantages of intraventricular catheters are that they measure global ICP, allow for therapeutic drainage of CSF, and are amenable to external calibration. Disadvantages are risk for infection, hematoma, or difficult insertion.
Microtransducer-tipped ICP monitors are placed in the brain parenchyma or subdural space either through a skull bolt, a burr hole or intraoperatively. They are almost as accurate as intraventricular catheters and have the advantages of lower infection and complication rates. The major disadvantages are an inability to drain CSF, no in vivo calibration and a small zero drift over time.
The initial treatment of a patient with an alteration in consciousness must employ management of the ABCs: airway, breathing, and circulation. Patients who are not responsive enough to protect their own airway require intubation to reduce the risk of aspiration of gastric contents. During intubation, hypoxia and hypotension should be avoided in brain-injured patients and a high suspicion of cervical spine injury should be maintained. A patient presenting with a GCS score 8 or less should be intubated. Mechanical ventilation should be used if necessary to provide adequate oxygenation and ventilation as guided by arterial blood gases. Patients presenting with hypotension and shock must be aggressively treated with fluids and vasopressors to maintain adequate perfusion. Sources of shock (septic, cardiogenic, hypovolemic) should be investigated and treated appropriately.
Regardless of the cause of acute decline of mental status, a number of general measures should be instituted. There should be a discontinuation of all drugs with potential toxicity to the central nervous system, if possible. Antipsychotic medications such as haloperidol or quetiapine can be used for management of severe agitation. Thiamine should be administered to patients with a history of alcoholism, malnutrition, cancer, hyperemesis gravidarum, or renal failure on hemodialysis in order to prevent the development of Wernicke encephalopathy.
In the event that a patient presents with history or examination findings suggestive of elevated ICP, immediate life-saving measures may be required prior to a more detailed workup with neuroradiological imaging or ICP monitoring. These situations generally rely upon clinical judgment and expert consultation with a neurosurgeon or neurologist is strongly recommended. An examination consistent with a critically elevated ICP (coma with GCS score < 8, unilateral or bilaterally fixed and dilated pupil(s), decorticate or decerebrate posturing and Cushing triad of bradycardia, hypertension, and respiratory depression) warrants immediate intervention while delaying additional diagnostic studies. In addition to standard resuscitation measures, elevation of the head above the heart (usually 30 degrees) to increase intracranial venous outflow, temporary hyperventilation to a goal Paco2 of 26-30 and administration of intravenous hyperosmolar therapy (either mannitol 1-1.5 g/kg or hypertonic saline infusion). Immediately following these measures, rapid evaluation of the underlying diagnosis by the patient history, detailed neurological examination and neuroradiological imaging should be pursued. Neurosurgical consultation should be considered for potential placement of a ventriculostomy as a means to assess ICP and potentially treat with CSF drainage.
If elevated ICP is present, therapy should be directed to maintain ICP less than 20 mm Hg. Interventions should be utilized only when ICP is elevated more than 20 mm Hg for more than 5 minutes. Transient physiologic elevations in ICP may be observed in the setting of coughing, movement, suctioning, or ventilator asynchrony that should not be targeted by therapy. During periods of elevated ICP, it is important to maintain adequate mean arterial pressure to ensure adequate cerebral perfusion pressure (which is mean arterial pressure – ICP).
Therapy with vasopressors should be targeted to maintain an adequate cerebral perfusion pressure (CPP = MAP − ICP), typically more than 60 mm Hg. Hypertension should generally only be treated when CPP more than 120 mm Hg. CPP more than 50 mm Hg is associated with cerebral ischemia.
Patients with elevated ICP should be positioned with the head elevated 30 degrees above the heart while maintaining the neck in neutral position without excessive flexion or rotation to maximize intracranial venous outflow.
When hydrocephalus (either obstructive or communicating) is discovered, a ventriculostomy may be utilized to reduce intracranial CSF volume and secondarily ICP. Controlled drainage of CSF at a rate of approximately 1-2 mL/min, at intervals of a few minutes until the goal ICP is reached (ICP < 20 mm Hg) or until CSF is no longer easily obtained is recommended. CSF drainage via an intrathecal lumbar drain is generally contraindicated in the setting of elevated ICP due to the risk of transtentorial herniation.
The use of mechanical ventilation to lower Paco2 to a goal of 26-30 mm Hg will induce cerebral vasoconstriction, decrease the cerebral blood volume, and rapidly reduce ICP. The effect of hyperventilation on ICP typically lasts for a period of hours prior to metabolic compensation. Therapeutic hyperventilation should be utilized only as an emergent intervention to temporarily control ICP and be replaced by other therapy modalities.
Osmotic diuretics reduce brain volume by creating an osmotic gradient from the brain parenchyma to the intravascular space, thus drawing free water from the parenchyma. Mannitol, prepared in a 20% solution, can be given as a bolus of 1-1.5 g/kg. Mannitol can be used in serial doses at intervals of every 6-8 hours at a reduced dosage on 0.25-0.5 g/kg as needed for continued elevated ICP. The onset of action is within minutes and the duration of effect varies widely from 4 to 24 hours. Serial measurements of serum sodium, serum osmolality, and renal function are necessary to prevent overdosage. Contraindications to the use of mannitol include serum sodium more than 150 mEq, serum osmolality more than 320 mOsm, or evidence of evolving acute tubular necrosis. In addition, mannitol frequently can induce hypotension and subsequently cerebral perfusion. Mannitol should not be used in patients with acute or chronic renal disease.
Bolus dosing of hypertonic saline (tonicity ranging 1.8-23.4 percent) can acutely lower ICP. The typical volume of hypertonic saline varies widely depending on tonicity, ranging from 30 mL of 23.4% to 1 L of 1.8%. Continuous infusion of hypertonic saline (1.8%-3%) to maintain hypernatremia may also be effective in controlling ICP. A meta-analysis of multiple clinical trials comparing the efficacy of mannitol to hypertonic saline for management of elevated ICP from a variety of causes (traumatic brain injury, stroke, tumors) found that hypertonic saline appeared to have greater efficacy in reducing elevated ICP, but clinical outcomes were not examined. Hypertonic saline should generally not be administered through a peripheral intravenous catheter.
Typically, patients with elevated ICP should be kept euvolemic and normo- to hyperosmolar. Administration of free water and hypotonic solutions should be strictly avoided and instead isotonic fluids should be used in all maintenance fluids and infusions. Serum sodium levels should be closely monitored and hyponatremia should be appropriately corrected.
Maintenance of adequate sedation can decrease ICP by reducing cerebral metabolic demand, ventilator asynchrony, and the sympathetic responses of hypertension and tachycardia. Adequate sedation typically requires the establishment of a secure airway. Infusion of propofol is often the drug of choice for sedation because it can be rapidly titrated, thus allowing for frequent neurologic assessments.
Fever increases brain metabolism, which increases cerebral blood flow and thus elevates ICP. Additionally, fever has been demonstrated to worsen brain injury in animal models. Therefore, aggressive treatment of fever, including acetaminophen and cooling, is recommended in patients with increased ICP.
Seizures, either convulsive or nonconvulsive, increase cerebral metabolism and result in ICP elevation. Aggressive treatment of seizures with antiepileptic therapy or infusion of anesthetics as well as continuous EEG monitoring is warranted. There is no clear evidence that prophylactic antiepileptic therapy is of any clinical benefit; however, consideration of prophylactic antiepileptic therapy is reasonable when high-risk mass lesions, such as those within supratentorial cortical locations, or lesions adjacent to the cortex, such as subdural hematomas or subarachnoid hemorrhage are present.
Glucocorticoids, typically dexamethasone, are reserved for the management of elevated ICP secondary to vasogenic edema secondary to intracranial neoplasm and infection. Typically, dexamethasone is dosed every 6-12 hours at a wide range of dosages. The use of glucocorticoids has been associated with a worse outcome in a large randomized clinical trial in traumatic brain injury and is no longer recommended. Glucocorticoids are not considered to be useful in the management of cerebral infarction or intracranial hemorrhage.
The use of barbiturates to control ICP is based on the drug’s ability to dramatically reduce brain metabolism and secondarily cerebral blood flow. Pentobarbital is most commonly used, with a loading dose of 5-20 mg/kg as a bolus, followed by 1-4 mg/kg/h. The barbiturate infusion is titrated based on assessment of ICP, CPP, and the tolerance of side effects. Continuous EEG monitoring is generally used, with titration to an EEG burst suppression pattern indicating appropriate suppression of cerebral metabolism. Barbiturate therapy is complicated and fraught with complications, particularly hypotension, adynamic ileus, reduced mucociliary clearance of the airway, and high risk of infections. In general, the use of barbiturates is reserved for elevated ICP refractory to all other treatment modalities.
The use of hypothermia to treat elevated ICP has been controversial for decades, and its use is not recommended as a standard treatment for increased ICP. Hypothermia decreases cerebral metabolism and secondarily reduces cerebral blood volume and ICP. When used, hypothermia is achieved by whole body cooling using surface or intravascular cooling devices to a goal of 32-34°C. Studies have demonstrated significant side effects, including cardiac arrhythmias and severe coagulopathy. Given the multiple uncertainties of the appropriate use of therapeutic hypothermia in patients with elevated ICP, this treatment should be limited to patients with intracranial hypertension refractory to other therapies.
Decompressive craniectomy is the surgical removal a large portion of the cranial vault to allow for the edematous intracranial contents to expand and subsequently reduce ICP. At the time of the procedure any mass lesion (neoplasm or hematoma) is also removed. Decompressive craniectomy has been considered a last resort; some evidence suggests that it does improve outcomes.
IMAGING OF THE CENTRAL NERVOUS SYSTEM
Imaging has become central to medical care in the past 25 years and is one of the fastest growing components of medical care expenditures in this country. In no field of medicine has imaging made more of an impact than in the neurosciences. Our ability to demonstrate anatomy and pathology noninvasively and to treat many pathologic central nervous system (CNS) processes utilizing minimally invasive techniques has grown immensely with improvements in imaging technology in the last few years.
Forty years ago, the only way to image the CNS was to replace cerebrospinal fluid (CSF) with contrast material (dye) or air via a lumbar puncture and take x-rays—a painful technique called pneumoencephalography—normal and abnormal CNS structures could be crudely outlined in this manner. Pneumoencephalography was abandoned in the late 1970s with the development of computed tomography (CT). Injecting dye directly into major blood vessels of the neck (cerebral angiography) has been available for three quarters of a century and allows exquisite delineation of intrinsic vascular pathology, but still does little to directly visualize the brain or spinal cord. While angiography is still performed today for both diagnostic and therapeutic purposes, the catheter is now placed via femoral arterial cannulation instead of directly into a neck blood vessel.
With the advent of CT scanning in the early 1970s, direct visualization of the brain was finally possible though resolving different normal brain structures and some pathologic processes remained difficult. Magnetic resonance imaging (MRI) first became available for clinical use in the early 1980s and has been the standard for evaluation of most CNS processes since the mid-1980s.
CT and MRI have continued to mature through the 1990s and into the 21st century. In addition to the introduction of lower radiation dose scanner capabilities on the most recent generation CT scanners, rapid scanning techniques on such scanners (“multislice” or “multidetector” CT scanners) now allow for collection of data reflecting cerebral blood perfusion, which can be collected during a 5-minute study. Similarly, such state-of-the-art scanners can be used to generate models of the major blood vessels of the brain (CT angiograms) lessening the need for more invasive intravascular catheterization in some patients.
MRI advances have been even more dramatic particularly with the release of higher field strength MR scanners (3.0 T) for clinical use in 2005. For example, the sensitivity of some of the newer MRI scanning techniques enables identification of physiologic changes in the brain minutes after ischemia occurs, a time frame that can potentially permit pharmacologic interventions that can affect clinical outcomes (eg, stroke). Functional MRI can assess eloquent areas of the brain near pathologic lesions (eg, tumors) that ideally should be avoided during surgery. MR spectroscopy (MRS) can identify lesion metabolites that can help discriminate among pathologic processes (eg, tumors vs. necrosis vs. ischemia vs. inflammation vs. infections). MRS can also identify metabolites accumulating in the brain in patients with congenital metabolic disorders. MRI can also be used to generate angiographic (MR angiography, MRA) images to evaluate blood vessels without injection of dye or the use of ionizing radiation.
Catheter (endovascular) angiography has been available in various forms for over 80 years, and has also continued to evolve over the last decade. Endovascular surgery—treating blood vessel abnormalities such as aneurysms or arteriovenous malformations (AVMs) through a catheter instead of with open surgery—is now commonly performed and has become the treatment of choice for the management of many lesions. More than half of aneurysms treated in the United States are currently managed endovascularly avoiding the need for craniotomy in the majority of those patients. Similarly, in the setting of an acute stroke due to an obstructing thromboembolus, in an attempt to reopen the involved vessel, a catheter can be placed in the region of the obstructing lesion and a clot mechanically removed through the catheter, or clot-dissolving (eg, thrombolytic) agents injected.
Plain radiographs (“x-rays”) are relatively inexpensive, universally available and can demonstrate osseous abnormalities such as fractures or gross destructive lesions (Figure 36–2). The main disadvantage of plain x-rays is that essentially all normal soft tissues (eg, all intracranial and spinal canal structures) and pathologic processes (eg, hemorrhage, infarctions, tumors, abscesses, herniated disks, etc) cannot be detected. Even many intrinsic osseous lesions are difficult to delineate. In fact, until 30%-50% of bone marrow trabeculae is replaced by a pathologic process (eg, tumor, infection), no osseous abnormality is seen on a plain radiograph.
Another limitation of plain radiographs is that all structures are superimposed on an single image as the x-ray beam passes through the entire head or spine (as opposed to the “slices” generated on CT and MR scans).
There is little role for plain radiographs for the evaluation of CNS disease as the interior of the head or spinal canal is not imaged. One exception is in patients with suspected child abuse. In these patients, in addition to other cross-sectional imaging (such as CT/MR which are performed to assess for intracranial injuries), plain radiographs should also be obtained as they may show subtle nondisplaced fractures not readily identified on other imaging tests.
Other roles for plain radiographs include assessing spinal motion (ie, lateral flexion/extension radiographs of the cervical or lumbosacral spine to assess spinal stability), localization of foreign bodies, and assessing intracranial or spinal structures where internal fixation hardware and/or other radiodense/ferromagnetic foreign material is present (which can limit the use of CT or MRI scanning).
2. Ultrasound of the CNS, beyond the neonatal period, is predominantly used in the intraoperative setting to evaluate ventricular morphology and underlying parenchymal pathology. Intraoperative ultrasound can be used to help position ventricular catheters during placement of shunts. It can also be used to guide intracranial and intraspinal tumor resections.
Outside of the operative setting, ultrasound has growing applications because it is noninvasive, portable, and does not involve radiation. However, an “acoustic window” is necessary for viewing the intracranial/intraspinal tissues meaning that overlying osseous structures usually must be removed before scanning can be performed (ie, portions of the skull or posterior spinal elements must be removed before the desired regions of the brain or spinal cord, respectively, can be evaluated).
Ultrasound has multiple applications in infants (usually such infants still have open fontenelles so no additional “acoustic window” to the brain needs to be created). It has become the imaging study of choice for the evaluation of premature infants to assess for intraventricular hemorrhage and/or hydrocephalus. In adults, intracranial Doppler examinations can be performed by scanning through sutures. For example, scanning through the temporal suture allows access to the circle of Willis. These examinations can be performed at the bedside for assessment of arterial vasospasm (eg, in the setting of subacute subarachnoid hemorrhage). Ultrasound can also be used in younger children to identify the conus medullaris.
CT scanning without or with intravenous administration of iodinated contrast material is performed utilizing a thin fan-like band of x-rays (ionizing radiation) that are generated by an x-ray tube that literally moves around the patient in approximately 1 second. The resultant image, which represents a “slice” of tissue, can be obtained with imaging thickness as thin as 1 mm. “Reformatted” submillimeter images can also be generated by the CT computer from the primary axially obtained CT scan data in any plane (sagittal, coronal, off-axis, etc). Three-dimensional images can also be created in this manner.
CT demonstrates most osseous abnormalities better than any other imaging test with the possible exception of nondisplaced, nondistracted fractures which are still often better seen on plain x-rays (Figure 36–3). Soft tissue lesions can also be detected with much better sensitivity than plain x-rays. However, resolving some similar, but not identical, normal soft tissues from one another, delineating normal from pathologic soft tissue, and evaluating certain areas of the brain which are limited by scanning artifacts can still be difficult with CT scanning which is why CT remains inferior to MRI for evaluation of most brain and spinal canal abnormalities.
When discussing CT images, the terms “density” and “attenuation” refer to the same process, namely, absorption of the x-ray beam. Areas of increased “density” have greater “attenuation” of the x-ray beam and result in more whitish areas on the CT scan image (eg, bones, iodine-based contrast material, acute blood, areas of calcification, and some foreign bodies). Areas of lower density have lower “attenuation” as they absorb less of the x-ray beam as it passes through the patient resulting in darker areas on the CT scan image (eg, fluid in the ventricles, gas, and fat).
CT image contrast can be adjusted at a workstation after scans have been obtained to highlight differences in the densities of different tissues.
Finally, the recent development of new methods of reconstructing CT data has resulted in significant reduction in patient radiation dosage exposure during many CT scans.
Myelography involves performing a lumbar subarachnoid puncture (or a lateral C1-2 subarachnoid puncture) and instilling less than an ounce of iodinated contrast material to opacify the spinal canal subarachnoid space which results in outlining cauda equina nerve roots and the spinal cord, and any process that impinges upon (or is within) the subarachnoid space. Such processes include extrathecal tumors, infections, herniated disk material, degenerative spinal changes, and also processes that directly involve the intrathecal structures (spinal cord tumors, vascular malformations, metastases, etc).
Myelography is no longer a primary imaging technique for evaluating the spinal canal having been replaced by MRI. It is currently reserved for “problem-solving” such as when the results of an MRI scan are not clear or when MR imaging is not possible (contraindications to MR scanning, internal fixation hardware limits evaluation by MRI, etc).
Myelography is essentially always followed immediately (within hours) by CT scanning to better delineate relationships of pathologic processes to the subarachnoid space.
MRI is an imaging technique that does not use ionizing radiation.
The physics of creating an MR scan are quite complex. Briefly, a patient is placed in a strong magnetic field (30,000 × that of the earth’s magnetic field). Radiofrequency pulses are transiently (milliseconds) applied to the patient to briefly perturb the patient’s water molecules by raising them to a slightly higher energy level (quantum mechanical model). After turning off the radiofrequency pulse, these water molecules rapidly return to their respective baseline states by giving off their recently absorbed energy. The rate at which these perturbed water molecules return to their respective baseline states can be measured by using extremely sensitive receiver coils in the MR scanner. As the rates at which these molecules return to their respective baseline states varies by local magnetic environment (eg, the local magnetic environment is different in the ventricular system as opposed to the lentiform nucleus, white matter, the eyeball, muscles, tumors, etc), these detectable differences among tissues can be localized in 3-dimensional space and used to create an image.
MR images are obtained which highlight different kinds of magnetic field differences between tissues. In general, most MR studies include “T1-weighted” and “T2-weighted” scans as part of the overall evaluation of the patient. MR scans take longer to perform than CT scans in part because T1-weighted (T1w) and T2-weighted (T2w) scans and often additional scans must be obtained separately. T1w images are distinguishable by the black appearance (“absence of MR signal”) of the CSF over the surface of the brain and in the ventricles. In contrast, on a T2w scan, the CSF over the surface of the brain and in the ventricles is white (Figure 36–4).
In general, T1w scans are best for delineating anatomy and areas of contrast enhancement. T2w scans are exquisitely sensitive to subtle changes in water concentrations (in both normal and pathologic tissues). These changes are seen in most pathologic processes (eg, strokes, tumors, infections). These subtle changes are manifest as increased signal (more whiteness) on T2w scans.
In the past 10 years, most MR studies include “FLAIR” (FLuid Attenuated Inversion Recovery) scans. In general, FLAIR scans can be considered super T2w scans on which normal fluid (eg, in the ventricles and subarachnoid spaces) has no signal (ie, black), and therefore, subtle pathologic process (which still appear white on the FLAIR scans similar to that seen on standard T2w scans) are easier to identify.
A gadolinium-based contrast agent can be injected intravenously to enhance the appearance of some pathologic processes such as those outside the blood-brain-barrier (BBB) or are intraaxial but do not have a functioning BBB.
MRI best delineates soft tissues both intrinsic and immediately extrinsic to the brain, can be performed in any plane (including nonorthogonal planes), and has no known side effects at the field strengths used for clinical studies. In addition to better demonstrating tissues than CT, MRI is not limited by many of the artifacts that limit evaluation by CT, particularly in the posterior fossa and spinal canal regions where CT can be severely limited. While not ideal for directly visualizing dense osseous structures (where many fractures, cortical erosions, and degenerative changes occur), MR is superb for detecting intrinsic (eg, bone marrow) osseous abnormalities (eg, metastatic disease, discitis/osteomyelitis, etc).
Cerebral angiography is performed by directly placing a catheter into the femoral artery, passing it cephalad in a retrograde manner up the aorta to the level of the aortic arch, manipulating it into either a vertebral or carotid artery and then further cephalad into the neck and even intracranially (Figure 36–5). The catheter is moved utilizing intermittent fluoroscopic guidance with small amounts of dye injected at selected intervals to confirm the location of the catheter. When in position, larger amounts of dye are injected and serial plain digital x-rays are rapidly exposed as the dye passes through the intracranial vessels in the distribution of the injected blood vessel. Over 100 images might be obtained during a single 8-second injection of contrast material. This procedure is associated with a 0.1%-0.5% chance of causing a stroke.
This technique best delineates intrinsic blood vessel pathology such as atherosclerosis, aneurysms, AVMs, fistulas, vasculitis, blocked vessels, and other intrinsic vascular disorders. It can be used to inject medications into specific blood vessel territories for diagnostic or therapeutic purposes. Catheters can be placed directly into aneurysms, vascular malformations, and recently occluded vessels for definitive therapy.
Endovascular (catheter) angiography is risky, expensive, requires complex equipment, and is performed by highly trained personnel. There are currently several alternatives to catheter angiography to evaluate for vascular lesions such as atherosclerotic vascular narrowing and aneurysms. Ultrasound (in the neck), CT angiography, and MR angiography are each useful alternatives in many situations (Figure 36–6).
Radionuclide Imaging (Scintigraphy) includes positron emission tomography (PET) and SPECT (single photon emission CT) scans which are molecular imaging techniques. Unlike other imaging techniques, these imaging modalities provide information beyond the structural appearance of normal and pathologic tissues. These techniques can provide physiologic information reflecting the functional state of tissues.
Low doses of radiotracers (positron emitters for PET scanning and single photon emitting isotopes for SPECT scanning) are used to label molecules or pharmacologic agents to image molecular interactions of biological processes in vivo. The nanomolar concentration of the radiotracer allows in vivo assessment of biologic processes without interfering with the process itself.
PET and SPECT cameras are able to detect, localize, and quantify regional distribution of radioactivity within the brain. Reconstruction techniques similar to those used in CT (eg, “filtered backprojection” and “iterative reconstruction”) yield 2-dimensional and 3-dimensional images of the brain.
The main advantage of a PET imaging over SPECT imaging is that PET utilizes “coincidence” detection, which is the ability to detect the simultaneous emission of 2 gamma rays that are generated when positrons annihilate when encountering negative electrons. Practically, this results in the greater spatial resolution of PET compared to SPECT. Both techniques can be used to study the same biologic processes.
The biological significance of measured radioactivity on PET and SPECT scans depends on the biological function of the molecule that is attached to the radioisotope. For example, radioligands are available to measure cerebral blood flow, blood brain barrier permeability, neurotransmitter synthesis, enzyme activity, receptor density, glucose metabolic rates, and gene expression, among other physiologic processes. Analysis of regional brain functions can be more specific by performing imaging both before and after specific pharmacological interventions, specialized motor or mental tasks (so-called “activation” studies), or therapeutic interventions, such as stem cell or gene therapy.
PET or SPECT imaging can also be used in the clinical arena for localization of epileptogenic foci, diagnosing dementing disorders, distinguishing between tumor recurrence and radionecrosis, and providing a chronologic record of disease progression (or response to therapy).
Finally, the same SPECT radioligands that are used for cerebral blood flow imaging (such as Tc-99m HMPAO) can also be used for bedside brain death studies using a standard planar gamma camera. Radioligands such as In-111 DTPA can be used intrathecally for radioisotope cisternograms for the clinical evaluation of patients with hydrocephalus or suspected CSF leaks. Radioisotope studies can also be performed to evaluate patency of CSF shunts.
Figure 36–2.
Lateral plain radiograph (x-ray) of the skull (ionizing radiation). Note the excellent delineation of the osseous structures, but poor delineation of soft tissues; specifically, no portion of the brain is imaged. Also, this study is not tomographic (ie, is not a slice) and therefore both the left and right sides of the head are superimposed.
Figure 36–3.
CT scan (ionizing radiation). This image represents a single section (“slice”) through the head (ie, it is a tomographic image; the left and right sides of the head can be separately delineated). The brain is directly imaged though resolving differences in intracranial structures can be difficult (eg, resolving gray and matter structures).
Figure 36–4.
MR scan (no ionizing radiation). Similar to CT, this image represents a single section (“slice”) through the head. The white matter and gray matter structures are more easily resolved that on a CT scan, and even some gray matter structures can be resolved (eg, putamina and globus pallidae).
Figure 36–5.
Angiogram (ionizing radiation). A catheter was placed in the right carotid artery in the neck and dye injected while imaging was performed in a front-to-back projection of the right side of the head. The bones are then digitally “subtracted” from the image. The exquisite delineation of the blood vessels of the right carotid territory are demonstrated, but no intra-axial (ie, brain soft tissues) structures.
Figure 36–6.
Aneurysm demonstrated by multiple imaging techniques. A. Catheter angiogram (ionizing radiation). B. CT angiogram (ionizing radiation). C. MR angiogram (no ionizing radiation). Note that the catheter angiogram (A), an imaging modality that is associated with a risk of causing a stroke, best defines this anterior communicating artery region aneurysm (arrow). However, the aneurysm is still well-seen on the CT angiogram (B), an imaging technique that is not associated with any risk of causing a stroke, and is also well-seen on the MR angiogram (C), an imaging technique that is not associated with any risk of causing a stroke and does not employ ionizing radiation.
MR data can be collected using software that images only moving tissues (ie, extravascular stationary soft tissue does not generate any MR signal on these studies while moving blood or CSF will generate signal). Using these techniques, images of blood vessels can be created with a sufficient level of detail that, in many cases, formal endovascular catheter angiography can be avoided (eg, demonstration of significant atherosclerotic disease in the region of the carotid artery bifurcation, surveillance imaging of a known aneurysm, etc). CSF flow evaluation can also be performed (eg, at the craniovertebral junction in Chiari patients).
Using rapidly applied magnetic field gradients, random molecular motion of water molecules can be converted to images and can be presented as “Diffusion” MR images. Rapidly applied gradients can also be used without or with contrast agent administration to assess cerebral blood perfusion.
Diffusion MR imaging is sensitive to early changes of cerebral ischemia, often on the order of minutes. In the setting of acute cerebral ischemia, MR diffusion imaging changes (which usually reflect acute irreversible ischemic change) and MR perfusion imaging changes (which reflect cerebral blood flow) can be performed. The MR perfusion and diffusion images can then be compared. As diffusion images usually represent permanent injury (infarction) and perfusion (blood flow) deficits are potentially reversible, if an MR perfusion deficit is more extensive than an associated MR diffusion deficit, it is possible that an area of perfusion abnormality without diffusion abnormality represents viable brain that is at risk to go on to permanent injury, but is not yet irreversibly injured (the so-called “ischemic penumbra”) and might benefit from aggressive therapy. Alternatively, if a diffusion deficit (representing a region of irreversible brain injury) is similar in extent to a perfusion deficit, then maybe the ischemic changes are permanent and there is no remaining “at risk” penumbra brain, and therefore no aggressive therapy is warranted.
Using standard MR hardware, MRS can be performed to evaluate brain metabolites. Normal brain tissue includes many metabolites the most important of which are N-acetyl-aspartate [NAA] (which is found in normally functioning neurons and decreases with neuronal injury), Choline [Cho] (a component of cell membranes which increases in any process that increases cellular turnover such as tumors, acute infections, etc) and Creatine [Cr] (a marker of cellular energy). Lactic acid can also be seen in ischemic cells (as a byproduct of anaerobic glycolysis), but is not present in detectable amounts on MRS in normal brain tissue.
CT can be used for guidance to percutaneously biopsy many lesions that previously required an open surgical procedure.
While rapidly infusing contrast material through a peripheral vein, using a high-speed multidetector CT scanner, data can be collected that reflects how well different portions of the brain are being perfused. In some patients, there are areas of underperfused brain which may manifest clinically with transient symptoms (eg, transient ischemic attacks); these regions might benefit from revascularization before the patient suffers irreversible injury (ie, a stroke). Evaluations similar to diffusion/perfusion brain MRI to assess for penumbra brain (see above) can also be performed.
While rapidly infusing contrast material through a peripheral vein, using a high-speed multidetector CT scanner, data can be collected that can be “reconstructed” with background data removed such that images similar to catheter angiograms can be created without risk of stroke. While intrinsic vascular detail is not as good as endovascular catheter angiography, in many patients, it is adequate to address the relevant clinical issue.
Small wires can be passed through an endovascular (ie, within a blood vessel) catheter and directly deposited into a saccular aneurysm where they form coils after release from the catheter; an aneurysm can be filled with these wire coils resulting in the complete obliteration of the patency of the aneurysm lumen eliminating the chance that such an aneurysm might rupture in the future and obviating the need for a craniotomy for placement of an aneurysm clip.
Instead of open surgery to address a narrowed atherosclerotic or dissected blood vessel, affected blood vessels can be treated via an intravascular catheter which first uses a balloon to dilate the narrowed portion of the blood vessel and then release a mesh stent to maintain the patency of the newly dilated vessel. Stents can also be placed at the base of wide-necked aneurysms allowing for the endovascular coiling of aneurysms that otherwise would not be technically possible.
An acute (within 6-8 hours of onset of symptoms) intraluminal occluding thrombus (clot) or thromboembolus can often be treated with intraarterial catheter placement within the blood clot with off-label (as of 2013) injection of a clot lysing agent (eg, tissue plasminogen activator [t-PA]) to reestablish blood flow to an affected vascular territory before permanent brain damage occurs. Alternatively, some intravascular clots can be captured and removed from the vascular system using specialized catheters [10].
CRANIOCEREBRAL TRAUMA
Head injury (traumatic brain injury [TBI]) is a leading cause of morbidity and mortality. The incidence of hospitalization for TBI is 75-200/100,000 population. TBI occurs among all ages, peaking in 15- to 24-year-old males. Head injury is very frequent in poly trauma patients managed by trauma surgeons or emergency physicians. Thorough familiarity of the basics of care is therefore highly desirable.
Motor vehicle accidents are the most frequent cause of TBI in the developed world, accounting for 30%-50% of all serious head injuries. Falls and recreational injuries account for about 10%-15% of TBI. Inflicted injury (assault) accounts for about 10%-20% of injuries in adult patients. Age and mechanism or injury are related, as assaults occur mostly to very young infants (child abuse) and to young adults ages 18-24. Falls are the most common source of head injury in patients over 80 years of age. Response to injury also appears to be age dependent. Young people, particularly young men, are more likely to suffer a brain injury, but the chances of dying from that injury are much higher in the elderly. Head injury is the leading cause of death among all patients suffering traumatic injury.
Head injury can be divided on clinical grounds into mild, moderate, and severe forms. About 80% of injuries are mild, including most concussions. Many patients with these injuries do not require hospitalization. Moderate and severe injuries account for about 10% each of the total injury burden and all of these patients are hospitalized. The death rate from head injury is estimated as 20-30/100,000.
Because recovery from moderate and severe injury is often incomplete, survivors of head injury and their care providers often must manage life-long disability.
Brain injury literature groups injury in several different and unrelated ways which may cause confusion. First, injury can be divided into primary versus secondary types. Primary injury occurs at the time of the brain injury or immediately thereafter. It includes the immediate deformative or concussive forces applied to the brain. Clinically, primary injuries include skull fractures, lacerations of the brain, and hemorrhage in and around the brain (which may take some time to fully accumulate). In contrast to primary brain injury, secondary brain injury denotes the brain’s response to injury, including, for example, loss of regulation of cerebral blood flow, cellular ischemic injury, and brain edema. Most of head injury research involves identification and interruption of these secondary injury pathways. Primary injuries can be induced by either focal or diffuse force application and by either linear or angular changes in momentum. The brain is much more sensitive to a diffuse, angular application of force than a focal, linear one. Experimentally, concussions occur at much lower total acceleration when an angular force is applied compared to a linear one.
Penetrating injury to the brain results in trauma both from the direct disruption of the brain cause by the projectile, as well as compression/decompression injury due to the passage of the bullet or other device. The energy that a projectile imparts to the head is directly proportional to the projectile mass and to the square of the velocity. Velocity therefore is usually the stronger determinant of the extent of projectile injury and the distinction between low- and high-velocity injuries is important.
Secondary brain injury describes the processes that occur in the brain in response to the primary brain injury. These occur from the sub-cellular to the macroscopic level. Calcium-dependent mechanisms, oxidative stresses from free radicals, and apoptotic mechanisms are all likely involved. Tissue ischemia clearly occurs after severe TBI, and in most cases cerebral blood flow (a marker for tissue perfusion) drops considerably in the early phases after severe TBI. The consequence of the microscopic injury cascade at a more macroscopic level is an increase in brain water content: cerebral edema. This occurs both through cytotoxic (cell injury and cell swelling) and vasogenic (incompetent vasculature) mechanisms, although likely the former mechanism is the more important. Brain edema acts as additional mass within the cranial vault that must be accommodated in managing intracranial pressure. The skull forms a rigid, protective covering for the brain. Any increase in the amount of material within the skull, such as a hematoma or edema, must be accommodated within the fixed volume of the skull, and will generally increase the intracranial pressure (ICP) (Figure 36–1). The intracranial compartment is subdivided into compartments by folds in the dura. The tentorium divides the cranial vault into a supra and infratentorial compartment while the falx cerebri divides the supratentorial compartment into right and left halves. This compartmentalization is of benefit after trauma by helping restrict the consequences of injury from impacting the other compartments. However, the compartmentalization and the protections that occur because of it are incomplete and in severe injuries, brain material will herniated out from its compartment of origin often producing specific clinical herniation syndromes.
The basics of management for patients with brain injury can be divided into initial resuscitation, primary neurologic survey, a search for lesions requiring immediate surgical management, and finally, identification and management of cerebral edema and increased ICP. These steps are often applied recursively as the patient condition may change frequently during injury course. The processes required to resuscitate, triage, and manage a concussion are less involved than for a severe brain injury, but the basic steps are similar.
Initial resuscitation for patients suffering brain injury is similar to that for all trauma victims. Management of the airway, breathing, and circulation (the ABCs) is paramount. While patients sustaining isolated mild TBI will usually maintain these functions, patients with multiple injuries, or patients with severe brain injuries often cannot maintain these critical functions without assistance. For example, hypoxia occurs in 30% of patients presenting with severe TBI, who often, because of their injury, cannot protect their airway. In addition to its obvious primary deleterious effects on the brain, hypoxia is also a strong stimulus to increase cerebral blood flow through vasodilatation of the cerebral vasculature. The additional volume of blood in the cerebral vasculature then adds measurably to the ICP after injury. Early endotracheal intubation to secure an airway and provide adequate ventilation is essential. All patients with severe brain injuries and many patients with more serious moderate brain injuries may require intubation, purely on the basis of a reduced ability to protect the airway and maintain ventilation. Intubation of a brain-injured patient should be performed with short acting pharmacologic agents. It is best to avoid the use of long-acting sedating medications and even the reflexive repetitive use of shorter acting agents in the prehospital and trauma bay settings. The loss of the neurological exam that results can impair or delay critical management decisions. Adequate analgesia and sedation are necessary for many trauma patients at intubation and during early resuscitation but the goal should be use the minimum necessary with repeated doses based on reassessment of patient status.
Restoring and maintaining an adequate blood pressure is also of critical importance. This should be accomplished by using intravenous fluids and blood as needed to restore a normal circulating blood volume, and by control of active hemorrhage. The use of pressor agents to support blood pressure may also be appropriate if hypotension persists despite an adequate circulating blood volume. Brain injury, unless it has progressed past a herniation event, is rarely the cause of hypotension, so that a standard search for the source of hemorrhage should accompany resuscitation as for any traumatic injury. Scalp injuries, which often accompany TBI, can be significant source of blood loss especially in children. Database research demonstrates that a single episode of hypotension following a brain injury doubles the risk of dying compared to patients who never have such an episode.
Once the ABCs have been addressed, the goal of the primary neurologic survey is a rapid, accurate categorization of the severity of the brain injury and a search for clinical evidence of large intracerebral hematomas that could require immediate evacuation. The Glasgow Coma Scale is a three component scoring system that is the main tool of the primary neurological survey (Table 36–1). To apply the scale, a patient is asked to give their name or description of what happened and to follow a simple command. Patients who are unresponsive to verbal requests should receive a brief, firm, noxious stimuli. An effective approach is to apply digital pressure to the trapezius muscle and observe for the response. Additional stimuli may be needed elsewhere to confirm the motor response. A patient can localize when their actions are purposeful and they attend specifically to a noxious stimuli, in an attempt to remove it. A patient is withdrawing when they do not attend to stimuli, but do act to move away from noxious stimuli in a nonstereotypic fashion. Decorticate and decerebrate posturing are stereotypic movements. The possible scores range from 3 to 15. Patients receive the better score when the left and right sides are different. Intubated patients may be given a score of “T” or their verbal score may be estimated from other communicative efforts that they make. The power of this grading scheme is that it is quick and accurate in terms of long term prognosis, assuming the examination is not clouded by sedatives or other confounding factors. Patients who score between 3 and 8 have severe TBI, 9-12 are categorized as moderate and 13-15 as mild closed head injury.
In addition to the determination of the GCS scale, the primary neurological survey includes an assessment of the pupils and gross motor function to assess for the presence of lateralizing signs of a large intracranial hemorrhage. These exams can also alert to the potential presence of a concomitant spinal cord injury. The mass of hematoma, depending on size and location, may produce uncal herniation with unilateral pupillary dilation and contralateral hemiparesis. These findings may indicate that a life threatening intracranial hemorrhage is present, which can be amenable to urgent evacuation. Depending on the situation it may be appropriate to assess other brainstem responses during the primary survey, but more often these are deferred until after an initial head CT scan is obtained. These brainstem reflexes can help to localization injuries (Table 36–3). Two additional brainstem reflexes, the Oculocephalic (doll’s eyes) and Oculovestibular (caloric testing) are not usually tested initial in traumatic settings because of potential exacerbation of cervical spine injuries (doll’s eyes) or basilar skull fractures (caloric testing).
Reflex | Afferent | Brainstem Level | Efferent | How Tested |
---|---|---|---|---|
Pupillary | CN II | Midbrain | CN III | Light shined in the eye, observed for pupillary constriction |
Corneal | CN V | Pontine | CN VII | Saline droped into the eye, observed for blink |
Gag | CN IX | Medulla | CN XI | Pharynx stimulated with ET tube or probe, observed for swallow/cough or aversion |
Once the patient’s airway, breathing, and circulation are safe, the severity of the head injury is determined and the primary neurological survey is completed, all patients with moderate and severe brain injuries should have a CT scan of the head. Rapid use of the head CT is also appropriate in many cases of mild closed head injury, especially when other risk factors, such as the use of anticoagulants, are present. Though the presence of a skull fracture significantly increases the chances of finding a lesion on a head CT, plain skull x-rays are not advised as a substitute to the head CT. MRI may at some point replace CT as the primary imaging tool for TBI, but this has not yet occurred. CT is quicker, safer for management of uncooperative patients, and safer for the environmental issues of loose ferromagnetic materials near, on or in the patient. MRI may be useful in assessing cervical and intracranial vasculature, and it may be helpful in more accurate prognostication of injury outcome.
The head CT is reviewed for the presence of surgically significant intracranial hemorrhage. In addition, there are specific radiographic markers that predict increased ICP (Figure 36–7). These include effacement of the basal and convexity subarachnoid spaces (so-called “cisternal effacement”), mass effect (compression/deformation of adjacent brain structures), and shifting of the brain contents from one side to the other causing “midline shift.” Patients with certain facial, skull base, and cervical fractures are at risk for cervical intracranial vascular injury and dedicated vascular imaging may be indicated. Conventional angiography, CT, and MRI-based angiography are all potential considerations in these situations. Conventional angiography is the gold standard for injury detection and offers the option of endovascular treatment; however, it is also time intensive and may separate the patient from the optimal critical care environment for a protracted period. CT angiography is convenient and rapid, but may miss some injuries. MRI angiography may be more sensitive but has the same downsides as MRI in other trauma settings. No highly regarded predictive tool exists, but risk factors that should prompt consideration of a vascular injury and subsequent vascular imaging include an unexplained neurological deficit, massive facial bleeding, or epistaxis, fracture involving the foraman lacerum of the skull base or foramen transversarium of the cervical vertebrae.
At the conclusion of the of the head CT scan, the clinical and radiographic information should be synthesized to formulate a plan either to operate to evacuate an intracranial lesion, to evaluate for intracranial hypertension with monitoring technology and clinical examination, or to observe clinically for worsening of neurological status with clinical examinations alone. Radiographic assessments, similar to clinical assessments, are often iterative with repeat imaging used regularly to assess for an alteration in status and optimal management plan.
There are several important clinical syndromes that herald herniation events within the brain. Each has both an imaging and clinical correlate. Immediate recognition and treatment of these is essential for patient survival. Herniation is the decompensated response to an increasingly large intracranial mass or unchecked cerebral edema. When the ICP or regional compartment pressure reaches a sufficiently high level, the brain tissue is displaced out of the compartment into the adjacent one. If all the intracranial compartments are under equally high pressure, the brain seeks to exit the calvarium through the foramen magnum. Subfalcine herniation occurs when part of the cerebrum is forced from one side to the other under the falx cerebri. This is evident radiographically by the degree of midline shift present on head CT or MRI. Usually the falx itself is shifted to some extent with the brain. In trauma, there is a general correlation between the degree of midline shift, the depression in the level of consciousness, and the severity of the injury. Rarely, the anterior cerebral artery can be pinched by the falx when there is subfalcine herniation. Uncal herniation occurs when the uncus (latin for “hook”) of the temporal lobe is shifted medially by a mass or swelling in the ipsilateral hemisphere. This is most likely to happen with temporal lobe masses because of the close proximity of the mass or swelling to the uncus. Anatomically, the uncus compresses the adjacent subarachnoid space, wherein lies cranial nerve III (oculomotor), resulting in loss of its parasympathetic fiber function to the ipsilateral eye and pupillary dilatation from unopposed sympathetic tone. With more severe or prolonged compression the extraocular muscle function of CN III is lost also, leading to a eye that is deviated interiorly and laterally (unopposed lateral rectus and superior oblique function). If the uncus is forced further medially, it compresses the cerebral peduncle producing contralateral hemiparesis. The clinical syndrome associated with these events therefore is an initial restlessness, then somnolence followed by a dilated ipsilateral pupil, and then contralateral hemiparesis. A somewhat confusing variation is the Kernohan’s notch phenomenon, wherein the entire brainstem is shifted by the uncus and the contralateral peduncle comes into contact with the opposite tentorial edge, leading to weakness that appears clinically on the same side as the pupillary dilation. This is relevant clinically, because if faced with the clinical contradiction of left pupillary dilatation and left hemiparesis, the left pupillary dilation is a stronger predictor of the ipsilateral nature of the cranial problem, than the left hemiparesis which would otherwise predict a right sided problem. Uncal hernation can have the secondary complication of compression of the posterior cerebral artery which runs near CN III. Patients are at risk for a PCA distribution stroke. Tonsillar herniation occurs when the intracranial contents, particular the contents of the posterior fossa, are forced out of the foramen magnum at the base of the skull. The tonsils of the cerebellum are pushed downward and compress the medulla oblongata, leading to respiratory depression and death. Radiographically, this is identified by the loss of CSF spaces around the brainstem and foramen magnum, as brain tissue is pushed into these spaces.
Cushing triad is the constellation of bradycardia, hypertension, and respiratory irregularity that often occupies a herniation event clinically. It is likely due to brainstem compression. The hypertension can be conceptualized as an attempt to protect the brain’s perfusion pressure from the high ICP. Intubation and mechanical ventilation often obscure the respiratory part of the triad, but the other two are observed regularly.
Skull fractures are the usually the result of focal application of force to the head. These are usually categorized as open or closed, depressed or nondepressed, and basilar or convexity varieties. Depressed fractures can tear the dura or lacerate the cortex of the brain. Depressed fractures greater than the width of the bone are usually considered for operative repair, particularly if there is an associated laceration of the skin. Skull fractures that are not depressed usually do not usually require repair. Basilar skull fractures (those fractures involving the bones at the base of the brain (parts of the sphenoid, temporal, occipital bones, and clivus) can damage the vasculature and cranial nerves, and can lead to a CSF leak and meningitis. Clinically, one may suspect a basilar skull fracture in the presence of Battles sign, which is retroauricular ecchymosis, or “raccoon eyes,” which is bilateral periorbital ecchymosis. Basilar and nondepressed calvarial vault fractures are usually managed conservatively, although their presence should prompt consideration of vascular and cranial nerve injury. Controversy exists as to the best management of fractures involving the inner table of the frontal sinus, or extending from the skull base into the ethmoid or other skull base sinus. There is the potential for the spread of infection through sinus communication with the epidural space. However, many such fractures heal spontaneously without complications, and management must be individualized.
An Epidural Hematoma (EDH) can occur with a skull fracture that lacerates an artery in the dura (Figure 36–8). The classic example is laceration of the middle meningeal artery by the temporal bone. The bleeding occurs on the outside of the dura and collects in and expands the potential epidural space between bone and dura. Because the source of bleeding is an artery, the hematoma can compress the adjacent brain to the point of serious injury or death. Not all epidural hematomas are caused by an arterial injury in the dura. Bleeding from a skull fracture or dural venous sinus can sometimes collect in the epidural space. These “venous epidural hematomas” are much less likely to produce life-threatening compression of the brain. Distinguishing between the two types is largely a matter of location (temporal vs. nontemporal), size (small vs. large), and rate of change (slow vs. fast). Because the primary injury in an isolated epidural hematoma does not involve the brain, the neurological outcome is excellent if the diagnosis and treatment are prompt. The classic presentation of a temporal epidural hematoma is a patient suffering a blow to the temple, and a brief loss of consciousness. This is followed by a lucid interval during which the patient appears to be neurologically well, as there has been little primary brain injury. During this time, the epidural hematoma either expands slowly enough for the compensatory mechanisms of the brain to maintain consciousness, or the hemorrhage stops temporarily. Subsequently, either due to rebleeding or exhaustion of the compensatory mechanisms, a rapid decline in level of consciousness follows from the mass of hematoma associated with the other elements of uncal herniation, including an ipsilateral CN III Palsy and contralateral hemiparesis. However, this classic sequence is only present in about 25% of patients with EDH. Many patients have no loss of consciousness with injury while conversely, about 20% decline after injury without the lucid interval. Epidural hematomas are usually diagnosed from CT imaging and have a lens-shaped (lentiform) appearance, as the dura is attached firmly to the skull at nearby sutures, tapering each end of the hematoma. CT images are also helpful to judge the impact on the hematoma on the brain, such as the degree of shift of the midline, or compression of adjacent structures. Management of an EDH is determined by lesion size, location, and time from injury to diagnosis. Generally, an EDH more than 1 cm in depth is considered for surgical removal, as are lesions in the temporal fossa where there is less room for further expansion before brain injury occurs. In some cases, an EDH is diagnosed two or more days after the injury that caused it. Since the bulk of hematoma expansion occurs within the first 24-36 hours, it is considered safe to observe patients with EDH of modest size (about 1-cm deep) when the diagnosis is made in a delayed fashion. The hematomas in many of these cases will reabsorb spontaneously. In contrast, a 1-cm-thick temporal epidural hematoma in a patient only 30 minutes out from injury should be strongly considered for emergent surgical removal of the hematoma prior to care of other injuries not immediately threatening to the airway, breathing, or circulation. The operative approach involves positioning the patient to gain access to the site of the hematoma. For a temporal epidural hematoma, a skin incision is made from the root of the zygoma extending into the ipsilateral frontal region in a reverse question mark fashion. As circumstances dictate, a burr hole can be placed in the temporal region, after incising the temporalis muscle before the skin incision is completed to allow blood to escape from the epidural space. However, in most instances, the blood is clotted and rapid completion of craniotomy must follow. The temporalis muscle is elevated and retracted anteriorly. Additional burr holes are placed as needed and a bone flap is elevated. The hematoma is removed. Lacerated dural vessels can be controlled with bipolar cautery or suture depending on size. The dural surface is inspected for an associated subdural hematoma, and intraoperative ultrasound may be useful as needed. The dura is then sutured to the bony margins of the craniotomy to prevent reaccumulation. When a hematoma has collected in the vicinity of a major dural venous sinus, such as the transverse or sigmoid, the sinus itself may be torn and the resulting hemorrhage that is very difficult to control. The outcome for a patient suffering even from a large EDH can be quite good if managed promptly.
Acute Subdural Hematomas (ASDH) are the result of trauma to the brain causing rupture of veins over the surface of the brain that transit from the cortical surface to the inner surface of the dura to reach the dural sinuses. When these vessels are injured, the blood collects between the dura matter and the arachnoid membrane, in the potential subdural space. The force required to shear vessels in this fashion can occur with a focal blow to the head, but is likely more common with diffuse, rotational force applications to the brain, as often occur in motor vehicle accidents. Except in the elderly, ASDH generally occurs in conjunction with fairly severe associated brain injury. Focal bruising of the brain is often present beneath the subdural hematoma. Most patients present with a significantly depressed level of consciousness and may have other findings related to the compressive mass of the hematoma. A subdural hematoma has more of a crescent shape to it on imaging studies, because there is no barrier to it spreading over the hemispheric surface of the brain. Associated intracerebral hemorrhage and edema is often evident beneath the ASDH (Figure 36–9).
Management of the acute subdural hematoma involves emergent operative removal by craniotomy for lesions more than 1-cm thick. A large craniotomy is generally required, and acute brain swelling during the surgical procedure must be expected. Unlike the situation in an EDH, the bleeding source in an ASDH is often difficult to discern. Diffuse hemorrhage arising from under the margins of the bone flap adjacent to dural venous sinuses, is often best managed with gentle packing of hemostatic materials rather than aggressive exploration. Medical management of elevated ICP and the underlying brain injury (see below) is an essential part of the patient’s care. Outcomes are often poor, with a mortality of 50%-90%. Survivors often have significant disabilities. Outcomes can be predicted by the admission Glasgow Coma Scale score and in certain cases medical care is likely to be futile and offered only with reservations.
In the elderly, the atrophy of the brain places the transiting veins under stretch and injury can occur with much less force. Subdural hematomas can present more chronically in such elderly patients, even several months after injury, with a several centimeter thick collection. Such chronic subdural hematomas should be thought of as a different injury from the ASDH (Figure 36–10).
In cases of chronic subdural hematoma, drainage of the hematoma either through a burr hole in the skull or via a craniotomy is indicated. Outcomes are much better than for ASDH, although recurrence and reoperation are common.
Coagulapathy, whether from anticoagulant medications, or as a complication of severe trauma, presents a special risk to TBI patients who are prone to sudden deterioration from hematoma expansion. Immediate, aggressive correction of coagulapathy is indicated for such patients.
Intraparenchymal contusions are common after trauma. These are hemorrhage mixed with brain and usually occur either at the site of a direct blow to the head, or at a point opposite the point of impact. This later phenomena is called a contra-coup injury and is the result of the pressure wave of force moving through the brain and impacting and rebounding from the skull opposite the impact site. Contusions often occur in anterior temporal lobes after frontal impact as the temporal lobes impact into the sphenoid wing anteriorly. The base of the frontal and temporal lobes is also a common site for contusions caused by the brain moving roughly over the irregular bony surfaces of the frontal and middle fossas. Posteriorly, this does not occur as the brain is moving over the smoother tentorium. The clinical presentation of such contusions is specific to their location in the brain, but they can also produce more widespread symptoms through their mass effect as discussed below in secondary brain injury. Intraparenchymal contusions should be distinguished from smaller hemorrhages associated with diffuse axonal injury (DIA) discussed below. There is certainly overlap in terms of size and location, but the typical intraparenchymal hemorrhages are more than 0.5 cm in diameter, and relate to either bony anatomy or force vector as described above. Management for these lesions is generally as conservative as the situation will allow because the hematoma is often intimately mixed with brain, some of which may still be functional. Lesions more than 25 cc are often considered for resection, but the relative eloquence of brain and the response to attempted medical management are often important considerations.
Penetrating brain injury usually produces a focal injury pattern specific to the site of penetration. High-velocity injuries such as those from a gunshot produce, in addition to the focal injury, a large area of cavitation, and hemorrhagic injury from the blast effect. Gunshot wounds that pass through the ventricular system, as a marker for the “middle” of the brain, are most often fatal. Management of less-severe penetrating injury revolves around managing the potential infectious complications of injury and repairing the breach to the skull.
Concussion is an immediate and transient loss of consciousness or normal mentation after head trauma, often associated with a period of amnesia. It is a very common injury. Sport injuries are a common source of concussions and young patients appear to be at highest risk. Concussion is most easily induced by sudden rotation of the head. The presumption is that the cerebral cortex is rotating around the more fixed midbrain and dienchephalon, producing disruption of input and outflow from the reticular activating system. Presentation after concussion can be surprisingly varied, with some patients displaying no loss of consciousness, but rather confusion and amnesia. The severity of concussion appears to be proportional to the duration of amnesia, particularly the anterograde (since injury) amnesia. Relying on consensus/expert opinion, several different criteria for diagnosis and grading systems for severity have been proposed. These systems use the duration of the confusion or amnesia and the presence or absence of a loss of consciousness to form a three-tiered scale of severity and are primarily designed to assist with return-to-play decisions for athletes. To the surgeon, the more typical concern is to decide whether a patient presenting with a concussion needs a CT scan of the head. Validated clinical decision rules stress that all patients with a Glasgow Coma Score of less than 15, those that are vomiting and those that are older than 60-65 years are at high enough risk to warrant a CT scan. Other factors warranting a CT for presenting concussed patients are severe headache, intoxication, persistent anterograde amnesia, a seizure with the injury, evidence of trauma to bone or soft tissue above the clavicle, and severe mechanism of injury such as autopedestrian or ejection injury.
Management for isolated concussions presenting for emergency room evaluation usually includes a period of observation for at least 2 hours, assuming the individual is neurologically normal. Dependent on any other injuries, the patient may be discharged to a responsible adult with written instructions to return for specific symptoms as listed for obtaining a head CT. Patient with abnormal CT scans are usually admitted for care. Long-term outcome is generally good with the majority of patients experiencing no long-term sequellae. However, up to 25% of patients report an increased incidence of headaches and memory difficulties even several months later. Postconcussive syndrome is a more severe version of this phenomenon that includes these and other symptoms such as depression, anxiety, emotional liability, insomnia, and fatigue. Treatment is largely one of reassurance and management of individual symptoms. Because athletes are often concussed, questions arise around when it is reasonable to return to play.
Traumatic Subarachnoid Hemorrhage is a relatively common finding in patients suffering severe TBI. Following injury, a relatively small amount of blood may collect in the subarachnoid space, often over the convexities of the brain, but also in the basal cisternal arachnoid spaces. Trauma is the most common cause of subarachnoid hemorrhage, not rupture of an aneurysm, so the report of subarachnoid hemorrhage after trauma should not automatically prompt a search for a ruptured aneurysm. A history of a neurological collapse before the trauma or more dense blood collecting in the basal cisterns or around the larger intracranial vasculature should prompt more concern for a cerebral aneurysm.
Diffuse Axonal Injury occurs when axons become sheared off at the boundary between gray and white matter during rapid brain acceleration or deceleration. The substance of the cerebral cortex is organized in to a series of alternating layers of gray and white matter (gray cortical mantel, subcortical white matter, deep gray matter nuclei of the basal ganglia, and white matter of the internal capsule). These layers have different tissue densities and when subjected to force in trauma, behave differently. The border between these two tissues is often a site of injury as these two layers accelerate or decelerate at rates according to their tissue properties. DIA is a common finding in severe injury, occurring in up to 50% of pts. Clinically, DIA can occur in varying degrees of severity. In minimal cases, a prolonged mildly concussive state of confusion and memory loss might occur. In more severe cases, the presentation is a depressed level of consciousness. Focal findings can occur if there are DIA hemorrhages in specific locations such as the internal capsule or brainstem. The appearance on head CT is one of multiple small (< 1 cm) hemorrhages, scattered throughout the brain at the junction of gray and white matter. Grading schemes that relate the severity of the CT findings to the eventual neurological outcome exist. Effacement of the basal cisterns and midline shift are examples of radiographic prognosticators of poor outcome after DIA. Management for patients suffering from DIA is primarily medical and focuses on prevention and management of secondary brain injury discussed below.
The medical management is largely directed at detecting and preventing secondary brain injury from seizures, systemic phenomena such as hypotension and hypoxia and intracranial hypertension.
The incidence of seizures following TBI is estimated at between 5% and 15%. The majority of these events occur within the first 7 days after trauma. A seizure has the possibility of increasing brain demand for oxygen and nutrients, at a point when the injury may limit the ability of the brain to respond in this fashion. Prophylactic anticonvulsants, usually phenytoin (dilantin), or more recently levetiracetam (Keppra), are reasonably used to prevent seizures after a moderate and severe TBI, when there is evidence of nontrivial brain injury on CT scans. However, experimental evidence only supports their use for the first 7 days after a TBI, and there does not appear to be benefit to continuing the prophylaxis beyond 7 days. Patients experiencing seizures outside of the immediate point of impact are candidates for therapeutic anticonvulsant use extending beyond the 7 day time frame, usually for several months or more, depending on whether the seizures recur.
Just as in the initial resuscitation, ongoing care of acute brain injury requires careful protection against hypoxia, hypotension, and hyperthermia. Each of these has the potential to increase ICP or further deprive the brain of adequate glucose or oxygen, or both.
Other than its impact on the neurological examination, secondary brain injury manifests itself clinically as cerebral edema and an increase in ICP. The Monroe–Kelly Doctrine states that given a fixed intracranial volume, consisting of brain, cerebrospinal fluid, and arterial and venous blood, any additional material must be accommodated by a decline in the amounts of the others, or a rise in pressure due to the increase in total intracranial material (Figure 36–1). When a mass is introduced into the cranial vault, CSF and later venous blood are displaced to make room for the mass while initially allowing relatively normal ICPs. However, these mechanisms are overcome and eventually the rise in pressure with increasing volume becomes exponential. The compensatory phase is very important clinically, as a patient may harbor a clinically important lesion while showing only modest symptoms of increased pressure in the brain. This model works well in trauma but is overly simplistic and does not explain clinical phenomena that occur over a longer time frame, such as might occur with chronic hydrocephalus or a brain tumor. In such cases, the compressibility of the brain, usually described as its compliance (change in volume for a given change in pressure) matters a great deal and brain pressures may be normal even in the face of a mass that, if it occurred acutely, would overwhelm the compensatory mechanisms mentioned above. Despite these limitations, the compartment model is very useful for managing cerebral edema and increased ICP. Left unchecked, these consequences of secondary injury lead to increased cerebral edema, increased ICP, compression and finally injury of adjacent brain and its vasculature, producing additional brain ischemia and tissue injury, cyclically generating more brain edema and further increased ICP. ICP is both a surrogate measure of the presence of cerebral edema and also a primary actor in ongoing secondary brain injury. Increased ICP, like hypoxia and hypotension, is a strong predictor of mortality and morbidity after head injury.
The concept of cerebral perfusion pressure (CPP) highlights the ability of elevations in ICP to reduce tissue perfusion:
where MAP is mean arterial pressure.
In adult patients, CPP of less than 70 mm HG is associated with worsened outcome. The equation also illustrates the importance of maintaining an adequate blood pressure in TBI management. Hypotension is among the strongest predictors of poor neurological outcome after TBI.
Detection of cerebral edema and intracranial hypertension is based on suspicion, radiographic features, and direct measurement of ICP. In severe head injury, as measured by the GCS score, the incidence of increased ICP is 50%-60%. Radiographic findings of concern include the presence of mass effect, midline shift, the loss of the evident pattern of sulci and gyri from displacement of CSF, the loss of distinction of the gray/white matter junction, and the effacement of the basal CSF cisterns. Increased ICP can occur in the absence of any of these findings, so in severely head injured patients, direct measurement of the ICP can still be indicated without concerning radiographic features. At present, it is unproven whether treatment directed by ICP is preferable to one based on CT and clinical examination. Nevertheless current guidelines from the Brain Trauma Foundation recommend ICP monitoring for:
All patients with GCS 3-8 and abnormal head CT;
GCS 3-8 with normal head CT but with hypotension or age more than 40;
Patients in whom the neurological exam cannot be assessed because of sedation or need for general anesthesia, if the suspicion of increased ICP is high.
ICP is measured in trauma by either introduction of a transducer into the brain parenchyma or placement of a catheter into the ventricles of the brain to measure the pressure of the cerebrospinal fluid in a minor surgical procedure. Complication rates of intracranial hemorrhage (2% vs. < 1%) and infection (10% vs. 2%) are higher for catheter-based measurement systems over parenchymal transducers, but only a catheter based system can drain CSF, a distinct treatment advantage. Parenchymal monitors are subject to drift in accuracy and may be inaccurate by 3-4 mm HG after 4-5 days. Catheter-based systems may occlude or be unreliable in the face of very compressed ventricles. In trauma, ICP is not measured by lumbar puncture as there is a risk of brain herniation from higher cranial versus lumbar pressures. Normal ICPs range between about 5 and 15 mm Hg at rest and vary with position and activity. Treatment thresholds after brain injury are typically more than 20 mm HG in adult patients, while children and infants likely require treatment at a lower ICP, although the precise numbers are not yet established. Measurement of the ICP allows calculation of the CPP. Managing brain injury patients based on CPP rather than ICP holds interest as a sufficiently high blood pressure should be able to perfuse the brain despite the ICP. However, clinical research suggests that while CPPs less than 70 mm HG in adult patients are associated with poor outcome, artificially raising blood pressure to supraphysiologic levels in an effort to overcome an increase in ICP, worsens, rather than improves outcomes. CPP measurements currently focus care on avoiding relative hypotension during management.
Treatment options for intracranial hypertension and cerebral edema are most easily understood with reference to the four compartment model of the brain noted above (brain, venous, arterial blood, and CSF). When patients present with elevated ICP, treatments available manipulate the volume of one of these compartments. At present, the selection and style of intracranial hypertension management is as much art as science. Few well-designed studies exist to directly compare different management strategies. Each treatment has risks associated with its use. General strategies include employment of less-risky strategies first and escalation as needed, and to apply methods directed at each of the compartments before applying multiple methods to the same compartment, although many therapies work on multiple parts of the model. The following section covers the treatment options in general but not rigid order of preference. A therapy from the end of the list would very rarely be used before one at the beginning, but that adjacent items in the list might be interchanged.
If blood is restricted from exiting the central nervous system, ICP increases as the venous compartment size is larger. In trauma patients, tight fitting cervical collars, a supine body position, and fighting against the ventilator all increase venous pressures. Loosening collars, elevating the head of the bed 30 degrees and minimizing ventilator pressures all improve venous drainage and lower ICPs. These measures have very little risk and can be employed widely.
Reducing the size of the CSF space can make more room for brain edema and lower ICP. This is done by draining CSF from the same ventricular catheter used to measure ICP. The risks of this therapy are infection and hemorrhage, and a capable surgeon must be available to place the catheter.
Besides caring for the patient’s comfort after an injury, sedation and/or chemical paralysis are important in reducing excessive brain metabolism that accompanies agitation from brain injury. This metabolism can be directly toxic in an injured brain, and obligates increased arterial and venous blood volume to supply the tissue with nutrients. In addition, sedatives/paralytic agents reduce fighting against the ventilator, reducing venous congestion. However, these agents reduce the ability to follow the neurological exam and have the side effect of hypotension when given in excess. Typically, agents such as morphine and ativan are used for analgesia and sedation, while muscular paralytics such as vecuronium are used to facilitate ventilation, but there is little evidence supporting the use of specific regimens with the exception of propofol, which should not be used in children. In general, shorter acting agents are preferable to long acting ones because of the desire to periodically examine the patient without their influence.
In theory, excessive brain water can be removed directly in some cases by establishing a favorable osmotic gradient for diffusion of fluid back into the blood stream. Mannitol, a sugar, and concentrated saline solutions (3% NaCl), do not cross the blood brain barrier and therefore provide such a gradient. Their effect appears to require an intact blood brain barrier. Experimentally, they draw water from less injured areas of the brain, rather than from the more injured areas. This reduces the overall brain volume, and can decrease the ICP, in the four-compartment model. In addition, experimental evidence suggests that a significant part of the ICP reducing effect of osmotic agents comes from reducing the viscosity of blood by altering RBC morphology. This allows delivery of more blood through smaller channels, permitting safe reduction in vessel caliber and, therefore, total intracranial blood volume. The adverse effects of these therapies include dehydration (and hypotension) in the case of mannitol, and nephrotoxicity from increased osmlolarity for all agents. In addition, some of the osmolar particles do make it across the blood brain barrier, and can pull water back into the brain if the therapy is withdrawn too quickly. Dosing regimens for these agents are variable but mannitol is usually used at 0.5-1 gm/kg per dose as often as every 2-3 hours. Dose of 1 gm/kg are given for impending herniation. NaCl 3% may be dosed either continuously at 1-3 cc/kg/h or as bolus doses of similar amounts. Serum sodium and osmolarity measurements should be assessed frequently. For mannitol, a serum osmolarity more than 320 mOsm/L appears to threaten toxicity and limit efficacy, while for hypertonic saline, serum osmolarities of 360 mOsm/dL or more have been reported without renal injury. In this area, the medical literature is limited.
The autoregulatory mechanisms of the brain rely in part on CO2 (or perhaps pH) concentrations in the blood. A high metabolic rate leads to increased CO2 production and acidosis. The natural response to this is vasodilatation, to remove waste products and increase the supply of metabolites. Clinically, artificially increasing the respiratory rate (and dropping the CO2) significantly decreases the intracranial blood volume by vasoconstriction. The downside risk is that if done to excess, the vasoconstriction produces ischemia. While hyperventilation was once widely practiced, it is now reserved for situations in which the brain injury has produced an excessive, as opposed to reduced degree of blood flow. This is relatively rare. However, it is import to manage ventilator settings to avoiding elevated CO2 levels (and reduced O2 levels). This avoids unnecessary vasodilation and the increased ICP that results. Arterial CO2 levels of 35 mm HG and O2 levels of 100 mm HG are the common therapy targets.
High doses of barbiturates reduce cerebral metabolism and experimentally protect against brain injury from the regional ischemia common in secondary brain injury (“the induced coma”). This reduced demand for metabolites decreases the blood flow requirements for adequate cell nutrition, and thus can decrease ICP. However, no clinical experiment has clearly shown that barbiturates improve outcome. The risk is that these agents can profoundly lower blood pressure. They must be used very carefully if at all.
In experimental settings, hypothermia appears to slow the destructive secondary injury pathways at a cellular level. This reduces the edema that comes from cell death. However, randomized trials in adult head injury have not shown benefit while pediatric trials are ongoing. In the published trial protocols, cooling is typically begun early in the hospital course, if not immediately, with target temperatures of 32-33°C maintained for several days after the initial injury. Complications associated with the therapy in the adult clinical trials have been an increase in infection rate, and serious electrolyte disturbances, particularly hyperkalemia.
Surgical management of cerebral edema involves enlarging the space available for swelling by removing a portion of the calvarium. The surgical technique is to remove a large portion of the skull over the more effected side (hemicraniectomy) or removal of large portions of both frontal bones (bifrontal craniectomy). The dura is generally opened and patched with either allograft or native periosteum. Clinical studies suggest that surgical decompression is effective in lowering ICP, but data regarding neurological outcomes are varied.
While many patients with mild traumatic brain injury return to their preinjury level of function, a significant number develop chronic symptoms of fatigue, memory impairment, headaches, and difficulty with concentration. In one study of prospectively followed injury victims, Thornhill, Teasdale, and colleagues reported that over 50% had some identifiable disability one year after injury, including both physical and mental impairments. These were severe enough to impact activities of daily living in one-third to one-fourth of patients. Predictors for poor outcome included age less than 40, and preinjury disability. The medical literature is mixed with regard to the longer term outcome. In a separate study by the Whitnall and coauthors, the overall rates of disability were similar at 1 year and 5 years from injury, but about 25% of patients had exchanged categories from good to disabled and vice-versa. Changes in depression, anxiety, and reported stress appeared to correlate strongly with category change, and only 7% reported the use of rehabilitation services by the 5 year postinjury point. Patients with moderate closed head injuries have more varied outcomes. Most recover to maintain their activities of daily living and even return to work or school. Detailed neurocognitive testing, however, often reveals deficits in executive function and memory. There are more consistent reports of chronic fatigue and headaches. Severe TBI is often a life altering event for both patient and family event. The prognostic implications of injury are often vitally important for family members making decisions about what degree of aggressive care to provide. As an average, perhaps 15%-20% of all patients with severe injury will make a good recovery, while more than 50% will either die or be severely disabled. Sadly, for some patients, the degree of injury and the poor likelihood of meaningful recovery make the provision of aggressive care an exercise in futility. Elderly patients, those more than 80 years old, with severe head injuries have a very poor prognosis. For patients with the worst prognostic features, such as older age, very low GCS or 3-5 without improvement with resuscitation, lack of pupillary response to light and associated chest or abdominal injuries with hypotension, the prognosis for meaningful recovery is very poor. Once the diagnosis and injury severity are confirmed by examination and imaging studies and the condition is unchanged despite resuscitation and withdrawal of all pharmacologic agents likely to be affecting the examination, a gentle but frank discussion of the situation is necessary and appropriate. However, generally, the younger the patient is, and the higher the presenting GCS score, particularly the motor GCS scores, even within the severe injury group, the better the chances of some degree of recovery. The physician would do well to remember that prognostic information represents a probability of an outcome not a certainty of it. While some families may appreciate knowing these details, others will more appreciate whatever kernels of hope can be provided under the circumstances.
SPINAL CORD INJURY
Traumatic spinal cord injury (SCI) is devastating. It primarily affects young people, and often results in significant disability or death. The median age at diagnosis of SCI is 37.6 years. The main causes in order of incidence are: motor vehicle collision, fall, violence, and sports injuries. Despite maximal medical and surgical therapy, the prognosis for significant recovery of a complete lesion is poor. Recent research into stem cell technology and other new modalities of therapy have not yet been effective in human clinical trials.
The cost to the healthcare system and to society is significant. A 25 years old with a high cervical cord injury (C1-4) is estimated to incur $741,425 in medical costs in the first year following SCI, and $132,807 for each year survived thereafter. In addition, the loss of wages and productivity for SCI patients averages $57,000 annually. With 12,000-14,000 Americans suffering SCI per year, the social and economic costs are significant.
The demographics of SCI have changed in the last 30 years. The median age of 37.6 years has increased from 28.7 years in the 1970s. This is a largely due to an increase in the incidence of falls causing SCI in patients more than 60 years of age. Though the relative incidence of both sports injuries and violent injuries has declined over the last 30 years, the larger decrease in sports injuries has placed it below violent injuries as a cause of SCI.
Treatment for SCI consists of acute and chronic management. Acutely, the ABCs (airway, breathing, circulation) must be secured, and the spine immobilized to prevent extension of injuries. Compressive lesions must be identified and the need for urgent surgical management determined. Methylprednisolone is currently a treatment option used widely in the initial phases of SCI, though it is associated with side-effects which may sometimes outweigh the potential benefits of its use. Other acute nonsurgical treatment options aimed primarily at minimizing secondary injury are modest induced hypothermia and hyperbaric therapy. The first would be based on the neuroprotective properties of hypothermia in brain injury but has not yet shown to have proven beneficial effects in humans with traumatic SCI. The second has shown to increase speed of neurological recovery but not an overall improvement in final outcome. Chronic management includes physical, and occupational therapy designed to maximize functionality. Specific therapy and improvement depends on the level and completeness of the injury.
Clinical findings in SCI depend on the level, mechanism, and severity of injury. Injuries can be classified as either complete or incomplete. A complete SCI refers to the lack of motor or sensory function below the level of the lesion. Incomplete lesions spare some degree of sensory and/or motor function below the level of the lesion. Incomplete lesions often result in recognized SCI syndromes based on the region of the spinal cord affected. The American Spinal Injury Association publishes a scale to further classify the severity of SCI (Table 36–4).
A =Complete: No sensory or motor function preserved in the lowest sacral segments (S4/5). |
B =Sensory incomplete: Sensory but no motor function preserved below the neurologic level including the sacral segments S4/5. |
C =Motor incomplete: Motor function is preserved below the neurologic level, and more than half of the key muscles below the neurologic level have a muscle grade less than 3. There must be some sparing of sensory and/or motor function in the segments S4/5. |
D =Motor incomplete: Motor function is preserved below the neurologic level, and more than half the key muscles below the neurologic level have a muscle grade greater than or equal to 3. There must be some sparing of sensory and/or motor function in the segments S4/5. |
E =Normal: Sensory and motor functions are normal. Patient may have abnormalities on reflex examination. |
Initial clinical findings include motor deficit, sensory deficit, and hyporeflexia. Initially, all reflexes below the lesion are lost including the bulbocavernosus, cremasteric, and abdominal cutaneous reflex. Over time, these reflexes may return, and the deep tendon reflexes become hyperreflexive due to the loss of descending tonic inhibition of the reflex arc. Initially, paralysis is flaccid, but eventually upper motor neuron signs develop, and a spastic paralysis results. If the lesion is in the high cervical region (C1-5), respiratory effort may be compromised due to the loss of innervation to the phrenic nerve. Loss of bowel or bladder function often occurs, and loss of rectal tone and sensation, as well as priapism may result. The loss of bladder control manifests as urinary retention, and developing urinary incontinence is typically overflow incontinence. Loss of anal sphincter tone and sensation results in leakage of stool and lack of awareness of bowel movements.
An important early finding that can occur in SCI is “spinal shock.” This refers to a drop in the systolic blood pressure with accompanying bradycardia, often to a level of 80 mm Hg systolic following SCI. This is due to the loss of sympathetic tone to the regions below the lesion and causes venous pooling and decreased venous return to the heart.
Chronic clinical findings in SCI are related to the long-term need for ventilatory support, immobilization, and need for catheterization. Pneumonia, urinary tract infections, and decubitus ulcers are common findings, and are often the cause of death in spinal cord injured patients.
Incomplete SCIs may demonstrate a variable pattern of sensory or motor preservation, though they can often be categorized into recognizable clinical syndromes, depending on the mechanism of injury and the portion of the cord affected.
Central cord syndrome (CCS) refers to a pattern of injury that affects the motor strength in the upper extremities more severely than the lower extremities. Sensory function is variable below the level of the lesion, and sphincter control is often affected. This usually occurs in older patients with spinal stenosis following a hyperextension injury. The central cervical cord is a watershed vascular territory that is thought to be disrupted in this syndrome. The spinal cord is somatotopically organized such that cervical fibers are more medial compared to fibers traveling to the lower extremities, resulting in the more severely affected upper extremities.
Anterior cord syndrome (ACS) results from the compression of the anterior portion of the cord by a herniated disk, bone fragment, or from occlusion of the anterior spinal artery. The corticospinal tracts and spinothalamic tracts are preferentially affected due to their more anterior location. The posterior columns are relatively spared. This results in loss of motor function and loss of pain and temperature sensation below the level of the lesion, with preserved proprioception, vibration, and pressure sensation. It is important to distinguish surgical from nonsurgical (ie, anterior spinal artery occlusion) etiologies in this condition.
Brown–Séquard syndrome occurs after spinal cord hemisection. It is usually the result of penetrating trauma occurring in 2%-4% of spinal cord injuries. Motor function and posterior column function (proprioception, vibration sense) is disrupted on the side of the lesion. Pain and temperature sensation is diminished on the contralateral side due to the crossing of the spino-thalamic tract in the spinal cord at or one or two levels above the entrance of the fibers into the cord.
Conus medullaris syndrome (CMS) results from injury to the sacral spinal cord. Symptoms include saddle anesthesia, loss of bowel/bladder function, and lower extremity weakness. It includes a combination of both upper and motor neuron signs.
Cauda–Equina syndrome refers to compression and dysfunction of the lumbo-sacral nerve roots. It is not a true SCI as it only affects the nerve roots and not the cord itself. The clinical syndrome is similar to CMS with saddle anesthesia, loss of bowel/bladder function, and lower extremity weakness, but findings are all lower motor neuron.
Initial physical examination in SCI focuses on the ABCs (airway, breathing, circulation). The spine should be immobilized to prevent further injury. Special attention must be focused on the airway in high cervical injuries as patients may require endotracheal intubation given injury to the nervous supply to the diaphragm. Blood pressure must be closely monitored given the possibility of spinal shock. This manifests as a drop in the systolic blood pressure and must be addressed immediately in order to prevent further cord ischemia.
In the awake patient after the ABCs have been attended to, a history focusing on mechanism of injury and detailed neurologic examination is undertaken in order to determine the level and completeness of the injury. Motor strength should be tested in all muscle groups and sensation should be tested with pinprick, and proprioception. Rectal tone and sensation should be tested with digital examination. Reflexes should be examined including the bulbocavernosus, cremasteric, and abdominal cutaneous reflexes. Careful palpation of the spine is important to evaluate for obvious step-offs or tenderness to palpation at all levels. The examination must be carefully documented and a neurologic level and evaluation of completeness of the lesion determined.
In the comatose patient, a complete neurologic examination is often difficult. In this situation, observation of spontaneous movements or movements to painful stimuli is important. Deep tendon reflexes should be examined and palpation of the spine should be undertaken to observe for obvious step-offs. Radiologic imaging is often required to adequately determine a level of injury and its etiology.
After a complete history and physical examination is performed, with the addition of radiographic imaging, the diagnosis of SCI is usually apparent. Radiographic imaging can help determine the mechanism of the injury, which is usually due to a fracture or subluxation of the bony spinal elements.
Some peripheral nerve lesions may resemble SCI, but these can usually be distinguished after careful examination and knowledge of the anatomy of the spinal cord and the peripheral nervous system. Peripheral injuries are typically unilateral and affect only lower motor neurons. Sometimes malingering and conversion disorders may mimic SCI. Serial examinations and inconsistencies in examination, in the setting of unremarkable imaging usually permits differentiation.
In the asymptomatic patient with no spinal tenderness, no distracting injury (eg, long-bone fracture), and no evidence of disturbed consciousness or intoxication, no radiographic imaging is necessary. Patients with spine tenderness, numbness, tingling, or obvious signs of SCI (eg, weakness, loss of bowel/bladder control) require radiographic imaging. The hallmark of radiographic imaging has been three-view cervical spine x-rays with AP/Lateral films of the thoracic and lumbar spine. Current recommendations of the American Association of Neurological Surgeons/Congress of Neurological Surgeons (AANS/CNS) recommend three-view cervical spine x-rays in conjunction with CT scanning of the cervical spine in patients with suspected SCI. With the advent of CT scanning with detailed coronal and sagittal reconstructions, CT scanning alone has replaced x-ray examination as the initial diagnostic study of choice in many centers (Figure 36–11). This obviates the need for multiple x-rays and diagnostic/treatment delay in the case of inadequate plain films. MRI is listed as an option in the diagnosis of SCI by the AANS/CNS as it can better detect ligamentous/soft tissue injury, though it often “overcalls” injuries that do not cause instability and may lead to prolonged and unnecessary immobilization. MRI is often reserved for patients for whom SCI signs and symptoms are present and no clear evidence is found on x-ray imaging or CT, or a herniated disk or other soft-tissue abnormality is suspected. MRI (particularly T2-weighted sequences) also clearly demonstrates compression of and/or signal change within the spinal cord (Figure 36–12).
In awake patients, clearance of the cervical spine consists of normal x-rays (or CT w/reconstructions), CT scan, and flexion/extension views or MRI obtained within 48 hours of injury. At this point, cervical immobilization may be discontinued.
Under current recommendations in obtunded patients, cervical spine clearance may be obtained following normal x-rays, CT, and dynamic flexion/extension films performed under fluoroscopic guidance, normal MRI obtained within 48 hours of injury, or at the discretion of the treating physician. However, with the advent of CT with sagittal and coronal reconstruction providing greater sensitivity for the detection of injury, and the propensity of MRI to “overcall” injuries, some centers clear the cervical spine in obtunded patient with normal x-rays and CT scans. Clearance of the cervical spine in this population is still cause for debate and recommendations are in flux. Further studies will elucidate the necessary and sufficient studies to definitively clear the cervical spine in this population.
In the patient with SCI the combination of x-rays with CT scanning has high sensitivity and identifies the vast majority of lesions causing SCI.
Initial treatment of SCI consists of securing the ABCs and immobilizing the spinal column. In the case of high cervical spine injury, the need for endotracheal intubation must be identified and if it is not immediately necessary serial arterial blood gas assessments should be monitored to evaluate for hypocapnia and progressive ventilatory failure. Spinal shock and the resultant decrease in blood pressure must be treated aggressively if it occurs. Volume expansion should be initiated promptly and decreased systolic blood pressure refractory to volume expansion should be treated with pressor therapy. The choice of pressors has not been conclusively defined, but typically a beta-agonist is followed by an alpha-agonist given the possibility of bradycardia in spinal shock.
Patients should be placed in a hard cervical collar and cervical immobilization should be ensured until clearance of the cervical spine, or definitive treatment has occurred. Patients should be placed on a board for transfers and log-rolled for movement until the thoracic and lumbar spine is cleared.
Other initial management considerations include placement of an arterial line to monitor blood pressure on a constant basis, and placement of a Foley catheter to decompress the bladder.
Methylprednisolone has been used in the acute phase of SCI based on studies that demonstrated motor improvement in patient groups that received methylprednisolone in the early period after SCI. However, due to the lack of demonstrated clinical significance of any improvement, and studies demonstrating side-effects of high-dose methylprednisolone, it is offered as an option by the AANS/CNS current guidelines with the knowledge that “evidence suggesting harmful side-effects is more consistent than any suggestion of clinical benefit.”
Following initial stabilization and imaging studies, the need for surgical intervention is assessed. Surgery has two main goals: decompression and stabilization. Surgery is employed on an emergent basis for incomplete lesions in the hopes of preserving or improving neurologic function, and on a nonemergent basis for complete lesions, as there is no demonstrated improvement in neurologic function for emergent surgery for complete lesions. Goals of surgery in this setting are to prevent cranial extension of injury, and to prevent progressive deformity. Choice of surgical approach for SCI is not standardized and is dependent on the location of the pathology. Current stabilization procedures typically involve instrumented fusion techniques, and may be approached via an anterior, posterior, or combined approach.
Cervical traction may be employed either alone, or as an adjunct to surgical therapy to attempt realignment of the spinal column. This is accomplished by fixing a halo ring, or specialized devices (ie, Gardner–Wells tongs) to the head, connecting this to a rope and pulley system, and adding weight to adjust the spine in the desired vector.
Chronic treatment of SCI focuses on rehabilitation and adaptation to permanent injury. Rehabilitation can often result in improved neurologic function in incomplete lesions and can help those with complete injuries become as functional as possible. Patients may require ventilatory support, tracheostomy, intermittent catheterization, frequent turning (to prevent decubitus ulcers), and functional accommodations such as wheelchairs and other devices aimed at improving functionality. Attention to long-term care issues can prolong the life and productivity of SCI patients.
Despite exciting research into novel treatments, SCI remains a devastating injury. Death in the acute trauma setting from SCI is 20%. Complete lesions that remain so at 72 hours are unlikely to improve beyond one level above the lesion in the long term. Patients with quadriplegia who have initial ventilator dependency have 5-year survival rates of approximately 33%. Incomplete lesions have a more favorable outcome. Among recognized SCI syndromes, CCS and BSS have the most favorable outcomes, with up to 90% of BSS and CCS patients being able to ambulate independently at 1 year. ACS patients have a worse prognosis, with 10%-20% recovering functional motor control. Causes of death in long-term SCI patients are usually due to cardiac, respiratory, or infectious causes—often related to the sequelae of SCI.
Current multidisciplinary approaches to SCI, including emergency department, medical, surgical, and rehabilitation staff provide the best therapy for patients with SCI. Despite this, SCI remains a devastating injury with high rates of mortality and permanent disability. Novel research and innovations will hopefully provide better outcomes for SCI in the future.
PERIPHERAL NERVE LESIONS
Familiarity with the pertinent aspects of peripheral nerve anatomy and physiology combined with focused history and physical examination aids in the management of peripheral nerve lesions. The history and physical examination may then be complimented by electrodiagnostic and radiographic studies.
Peripheral nerves are composed of varying combinations of sensory and motor axons. An axon is a long projection from a nerve cell body that is bounded by a cell membrane as well as a basement membrane. Some axons are surrounded by sheaths of myelin, a fatty substance secreted by Schwann cells. Myelin insulates the axon, thereby increasing the velocity of neurotransmission. The axon is, in turn, surrounded by a layer of connective tissue called endoneurium. Axons travel together in bundles called fascicles, each of which is covered by another layer of connective tissue called perineurium. Fascicles are grouped together to form a peripheral nerve, which is surrounded by a final layer of connective tissue called epineurium.
A peripheral nerve is comprised of fibers from more than one spinal nerve root and each spinal nerve root contributes fibers to more than one peripheral nerve. Spinal nerve lesions manifest as radiculopathies with blurred sensory disturbances, while peripheral nerve lesions demonstrate sharply demarcated sensory disturbances. Spinal nerve lesions results in mild to moderate weakness in muscles supplied by one spinal nerve, but by more than one peripheral nerve. Peripheral nerve lesions manifest more severe muscle atrophy and weakness in muscles supplied solely by the peripheral nerve.
Common etiologies of acute peripheral nerve injury include penetrating trauma, blunt trauma, traction, fractured bones, or compression from hematomas. Minor peripheral nerve injuries arise from blunt trauma that temporarily compresses or stretches a nerve, but leaves its axons intact (neurapraxia). In such cases, axonal transport may be temporarily impaired, but Wallerian degeneration, or the death of axons distal to the point of injury, does not occur. These injuries generally recover spontaneously over the course of days to weeks. Slightly more severe injuries may interrupt axons and their myelin sheaths while leaving endoneurium intact (axonotmesis). In these cases, Wallerian degeneration inevitably follows. Axonal regeneration may occur spontaneously, however, guided to areas of previous innervation by intact endoneurial tubes. With this type of injury, there is a good prognosis for spontaneous functional recovery, with axonal regeneration occurring at a rate of about 1 mm/d or 1 inch/mo.
Peripheral nerves may be severed cleanly (neurotmesis), as in the case of iatrogenic scalpel injuries during surgery. If the divided ends of the nerve remain in proximity, regeneration can occur via axonal sprouting from the proximal stump. These axonal sprouts may bridge the gap to the distal stump, propagating through preserved endoneurial tubes at a rate of 1 mm/d. Severe crush injuries may create internal damage to a peripheral nerve without completely transecting it. Such injuries disrupt axons and their endoneurium and disturb the organization of fascicles within the nerve. In these cases, fibrous scar tissue may form within the macerated nerve which can block the regeneration of axonal sprouts. A tangle of axonal sprouts contained in fibrous scar tissue is called a neuroma. Neuroma formation acts as a barrier to spontaneous peripheral nerve regeneration.
A careful clinical history and a meticulous neurological examination are paramount in determining which peripheral nerves have been injured and the type of injury present. The type of trauma will generally suggest whether or not the nerve is in continuity. A penetrating injury with a sharp object, such as a knife, suggests a clean transection that is amenable to immediate surgical repair. Nonpenetrating trauma or a stretch injury is more suggestive of nerve continuity.
Determine the timing of motor and sensory deficits may also aid in the assessment of the nerve injury. For example, in the setting of a penetrating sharp injury, an immediate deficit at the time of the injury would suggest direct involvement of the peripheral. However, a delayed deficit would suggest an enlarging adjacent lesion such as a hematoma or pseudoaneurysm.
The physical examination includes inspection, observation, evaluation of the relevant vasculature, range of motion assessment, and neurological examination. A laceration, fracture, or bruising/abrasions may suggest the site of an underlying nerve injury. A Horner’s sign (ptosis, meiosis, anhydrosis) is suggestive of a proximal T1/lower trunk brachial plexus lesion. An impaired range of motion can make assessment of strength difficult to evaluate accurately. An elevated hemidiaphragm is suggestive of a phrenic nerve injury.
The sensory and motor findings associated with acute peripheral nerve injury vary widely, depending on which particular nerve is injured. Pain may also be a symptom, but it usually develops in a delayed fashion. Pain can occur as a result of neuroma formation, where it is often associated with a tender lump in the area of injury. Neurogenic pain may also develop because of a disturbance in the processing of pain signals. This type of pain, when associated with autonomic hyperfunction, is referred to as complex region pain syndrome (formally known as causalgia or reflex sympathetic dystrophy); it is notoriously difficult to treat. When neurogenic pain is associated with nerve root avulsion it is known as deafferentation pain. Deafferentation pain often responds well to surgical intervention via dorsal root entry zone ablation.
In the diagnosis of acute peripheral nerve injury, EMG and nerve conduction studies are generally not useful until at least three weeks after injury. Nevertheless, it is important to obtain baseline electrodiagnostic studies as they are important for monitoring recovery. In the case of brachial plexus injury, it is useful to obtain an MRI scan or CT myelogram to look for pseudomeningocoeles in the vicinity of the nerve roots, which would indicate nerve root avulsion.
Certain peripheral nerve injuries are associated with traumatic fractures of specific bones. For example, the radial nerve is particularly vulnerable to injury from fractures of the humerus. Traumatic injuries of the radial nerve classically occur with fractures of the shaft of the humerus, at the level of the spiral groove. Such injuries result in weakness of wrist extension, finger extension, and thumb extension, as well as numbness over the radial aspect of the dorsal surface of the hand. In this type of injury, elbow extension is not affected, since muscular branches to the triceps are given off proximal to the spiral groove.
Trauma to the brachial plexus can cause a wide array of neurological signs and symptoms. Clinical manifestations are determined by the location of the lesion within the brachial plexus as well as the severity of the injury. Erb-Duchenne palsy is a well-described condition involving injury primarily to the upper trunk of the brachial plexus (derived from C5 and C6 nerve roots). It typically results from a stretch injury such as traction on the arm at the time of birth, or a fall that forcefully separates the head from the shoulder. The resulting deficits to the deltoid, biceps, rhomboids, brachioradialis, supraspinatus, and infraspinatus, leave the arm hanging to the side, internally rotated and extended at the elbow. This posture is often called the “waiter’s tip position.”
In acute trauma, when unilateral limb findings are present, it is important to differentiate acute radiculopathy from peripheral nerve injury. A thorough neurological exam is critical. Several general principles should be considered. Radiculopathy is often accompanied by neck or back pain, which tends to radiate down an arm or a leg. Also, the sensory findings of radiculopathy tend to be blurred, reflecting the overlapping nature of dermatomes, while sensory findings in peripheral nerve injuries are sharply demarcated. Weakness from radiculopathy occurs in muscles innervated by one spinal nerve, but by more than one peripheral nerve. Thus, it is often only partial weakness, since nearly all muscles are innervated by more than one spinal nerve.
One crucial task in diagnosing acute peripheral nerve trauma is to rule out ongoing neural compression. Acute trauma to a peripheral nerve usually results in maximal deficits at the time of injury. A peripheral nerve deficit that progresses should raise a red flag and initiate further workup. Immediate surgical exploration should be considered in order to address compressive lesions such as expanding hematomas or growing traumatic pseudoaneurysms. Sources of ongoing neurologic compression should be removed as soon as possible.
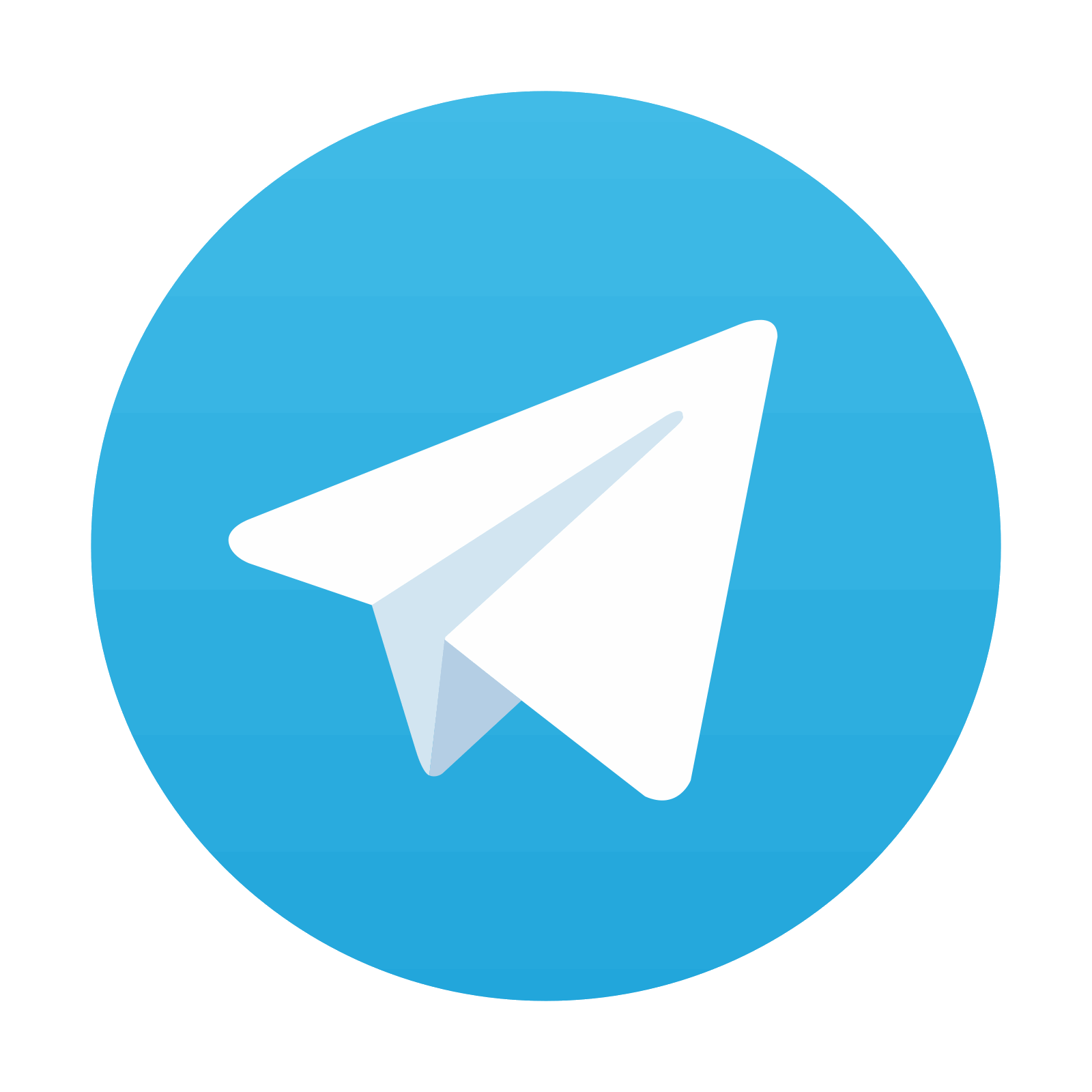
Stay updated, free articles. Join our Telegram channel
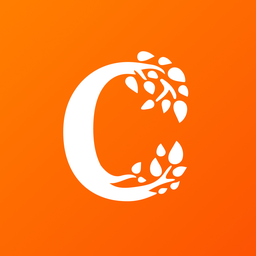
Full access? Get Clinical Tree
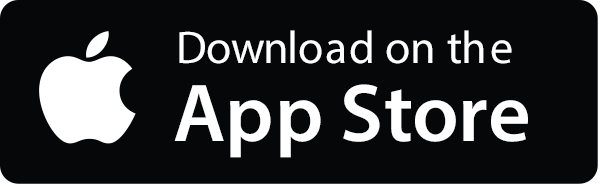
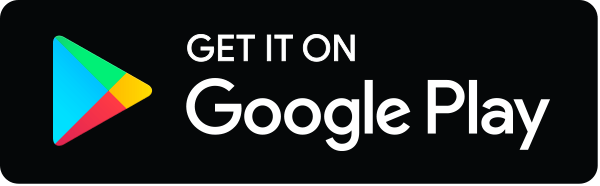